Translate this page into:
Chloroquine chaos and COVID-19: Smart delivery perspectives through pH sensitive polymers/micelles and ZnO nanoparticles
⁎Corresponding authors at: ICAR-National Research Centre on Equines, Hisar-125001, Haryana, India. amanuja@rediffmail.com (Anju Manuja), bmanuja.nrce@gmail.com (Balvinder Kumar)
-
Received: ,
Accepted: ,
This article was originally published by Elsevier and was migrated to Scientific Scholar after the change of Publisher.
Peer review under responsibility of King Saud University.
Abstract
Abstract
(Hydroxy)Chloroquine is a well known zinc ionophore besides possessing antiviral effect. Zinc oxide nanoparticles have been reported to possess antiviral activity. The possible mechanism as an antiviral for both has been discussed in depth. Adverse effects inflicted by (hydroxy)chloroquine and its controversial role in COVID19. Perspectives discussed for smart delivery options through pH responsive copolymer micelles/drug encapsulation to address this issue.
Abstract
The global pandemic of COVID-19 had a consequential impact on our lives. (Hydroxy)chloroquine, a well-known drug for treatment or prevention against malaria and chronic inflammatory conditions, was also used for COVID patients with reported potential efficacy. Although it was well tolerated, however in some cases, it produced severe side effects, including grave cardiac issues. The variable reports on the administration of (hydroxy)chloroquine in COVID19 patients led to chaos. This drug is a well-known zinc ionophore, besides possessing antiviral effects. Zinc ionophores augment the intracellular Zn2+ concentration by facilitating the zinc ions into the cells and subsequently impair virus replication. Zinc oxide nanoparticles (ZnO NPs) have been reported to possess antiviral activity. However, the adverse effects of both components are also reported. We discussed in depth their possible mechanism as antiviral and smart delivery perspectives through pH-sensitive polymers/ micelles and ZnO NPs.
Keywords
ZnO nanoparticles
COVID 19
Chloroquine
pH responsive
Micelles
Polymers
1 Introduction
Coronaviruses are enveloped, single-stranded positive-sense RNA viruses that cause diseases in mammals and birds. In humans, coronaviruses cause respiratory disorders ranging from mild to lethal infections. They became a matter of concern for the whole world due to their lethality and transmissibility from person to person. Transmissible gastroenteritis virus in swines, bovine coronavirus in cattle, and infectious bronchitis virus in fowls are of veterinary significance (Weiss and Navas-Martin, 2005). The world observed the infection in humans as “Severe-acute-respiratory syndrome (SARS-CoV)”, “Middle-East-respiratory syndrome coronavirus (MERS-CoV)”, “SARS CoV2” in 2002, 2012, 2019 respectively (Rathore and Ghosh, 2020; Wang et al., 2013). SARS and MERS were both responsible for high mortality rates. Involvement of multi-organs and variable symptoms has been reported in SARS CoV2/COVID-19 affected patients. The most common manifestations reported in COVID-19 patients are fever, dry cough, and tiredness (Wu et al., 2020) and the people showing only these signs generally recovered without hospitalization. However, diabetes is associated with the severity of the disease in COVID-19 (Huang et al., 2020). Severe symptoms include shortness of breath, loss of speech, loss of movement, hypoxemia, shocks, heart injury, renal injury, etc (Mehta et al., 2021). In addition to this, bacterial and fungal infections may also attack COVID-19 patients. Currently, there is no treatment for coronavirus, and it is based on symptoms only. However, according to a recent study, three new oral antivirals (molnupiravir/fluvoxamine/paxlovid) are effective in lowering the mortality and hospitalization rates of COVID-19 patients (Wen et al., 2022).
Chloroquine phosphate, an aminoquinoline, is an old drug known mainly for the treatment of protozoan disease, malaria, and lingering inflammatory ailments (rheumatoid arthritis (RA), systemic lupus erythematosus, etc.). Another related drug, hydroxychloroquine which is also derived from quinoline molecules, is meant for similar clinical conditions. Hydroxychloroquine differs from chloroquine owing to the hydroxyl group (OH) and is preferred over chloroquine in its use against malaria & viral diseases due to its low ocular toxicity. Both these drugs demonstrated inhibition of SARS COV2 in vitro (Wang et al., 2020; Liu et al., 2020; Yao et al., 2020; Vincent et al., 2005). The drug has shown noticeable efficacy against COVID-19 associated pneumonia in China's clinical trials (Gao et al., 2020; Jie et al., 2020; Gautret et al., 2020). Hydroxychloroquine and chloroquine phosphate were consequently advocated for prophylactic and therapeutic usage for COVID-19 patients by the Indian Council of Medical Research, India and the National Health Commission, China (Gautret et al., 2020; Jie et al., 2020). (Hydroxy) chloroquine emerged as a potential drug for the treatment and prevention of the SARS COV2 infection in many countries (China, France, USA, and India) (Gao et al., 2020; Jie et al., 2020; Gautret et al., 2020). Initial medical information confirmed that (hydroxy)chloroquine avoided the aggravation of pneumonic cases and, reduced the viral load in SARS COV2 infected patients. Hydroxychloroquine in combination with azithromycin was granted restricted emergency-use approval by US-FDA to deal with COVID-19 patients (Gautret et al., 2020). A total of 290 clinical trials have been done on hydroxychloroquine, of which 96 have been completed (http.//Clinical trials.gov, 2022). Forty studies on hydroxychloroquine were terminated; 8 studies were suspended; 42 studies had been withdrawn, and 57 were considered as unknown status. Out of 96, only two studies were found to be associated with zinc. Clinical trials conducted on hydroxychloroquine in a few countries yielded variable outcomes. Some studies have indicated the benefits of chloroquine/ hydroxychloroquine as therapeutics for COVID-19, whereas other trials have reported adverse effects that have been discussed in detail elsewhere (Bansal, 2021). The World Health Organization suspended global trials of chloroquine, and its derivative hydroxychloroquine in treating the global pandemic of COVID-19 given its potentially toxic effects, but later resumed the solidarity trials for the drug. The emergency authorization of chloroquine/hydroxychloroquine use was then suspended due to a lack of efficacy in clinical trials by the FDA and other regulatory agencies. Lancet editors provided an expression of concern for the retracted study “Hydroxychloroquine or chloroquine with or without a macrolide for treatment of COVID-19: a multinational registry analysis” (Lancet, n.d.) which failed to verify a positive impact of hydroxychloroquine or chloroquine, either alone or in combination with a macrolide, on COVID-19 in-hospital outcomes. When used to treat COVID-19, each of these medication regimens was linked to a lower rate of in-hospital survival and a higher incidence of ventricular arrhythmias. Four repurposed antiviral medications, including hydroxychloroquine and three others (remdesivir, lopinavir, and interferon β-1a), were suggested for mortality studies by World Health Organization expert committees in patients who were hospitalized with COVID-19 (WHO Solidarity Trial Consortium, 2021). The inefficacy of hydroxychloroquine in those trials was not due to the lack of antiviral activity of the molecule, which was repeatedly and reproducibly shown by many groups throughout the World (Liu et al., 2020). The reasons for the lack of efficacy of hydroxychloroquine in the clinical trials are two fold: (i) Cells from the lower respiratory airways become insensitive to chloroquine/hydroxychloroquine due to activation of transmembrane serine protease 2 (TMPRSS2) (Ou et al., 2021). Therefore, it is expected to become inactive in the later stages of the disease when pneumonia is developed. ii) The virus-inhibitory concentrations only in part match the tissue and, especially, plasma concentrations of the drug. Therefore, mixed results were expected in the early stages of COVID-19, when the virus remains in the nasopharynx (Tarek and Savarino, 2020). The further description about the efficacy of the drug against the virus is given in the next section under possible mechanism of (hydroxy)chloroquine action against SARS COV2.
2 Possible mechanism of (hydroxy)chloroquine action against SARS COV2
Numerous phases in the coronaviral lifecycle have been proposed to be restricted by (hydroxy)chloroquine, but it's inhibitory effect on viral entrance as a lysosomotropic drug is well described. (Hydroxy)chloroquine comprises an ‘amino group’ connected to a ‘quinoline ring’, which are feeble, susceptible ‘diprotic bases’ that possibly accrue within intra-cellular acidic cubicles including lysosomes (Kaufmann and Krise, 2007). It augments the lysosomes’ pH and causes enlargement, vacuole formation, and impaired lysosomes’ function (Yoon et al., 2010). Both receptor activation and fusion activation by proteolytic processing of the S glycoproteins are necessary for coronavirus entrance. SARS CoV2 requires the angiotensin-converting enzyme 2 receptor (ACE 2) for entrance and the serine protease TMPRSS2 for S protein priming. The drug increases endosomal and lysosomal pH, which in turn prevents cathepsin L from functioning as one of coronaviruses' entrance factors. It effectively interferes with the glycosylation of its host receptor, ACE 2, suggesting similar effects of the drug against this virus at its point of entry into the cells (Hoffmann et al., 2020). These drugs, after accumulating within the intracellular compartment and raising the endosomal pH, rapidly transport into acidic vesicles, and impede terminal glycosylation. Consequently, this hinders the fusion procedure and puts off the release of viral RNA particles, therefore inhibiting the multiplication of viruses intracellularly, thus restricting the virus's survival (Al-Bari, 2017; Akpovwa, 2016). The first pathway is dependent on the endosomal protease cathepsin and susceptible to the drug, while the second process relies on TMPRSS2, which is suggested to be unaffected by hydroxychloroquine (Ou et al., 2021).
The literature reports that chloroquine inhibits pH-dependent multiplication of human immunodeficiency, influenza, dengue, Japanese encephalitis, West Nile and Zika viruses and thus restricts their infection (Tsai et al., 1990; Ooi et al., 2006; Farias et al., 2013; Boonyasuppayakorn et al., 2014; Zhu et al., 2012; Delvecchio et al., 2016). The drug also diminishes the expression of phosphatidyl inositol-binding clathrin assembly (PICALM), an abundant protein in clathrin-coated pits (Hu et al., 2020). PICALM regulates the size of the clathrin cage which senses and drives membrane curvature, thus controlling the pace of endocytosis.
Literature also suggests that drug acts by hampering quinine reductase 2, which is required for sialic acid biosynthesis (Kwiek et al., 2004). Sialic acid was involved in the binding of MERS-CoV and hCoV-OC43 and its access into host cells, and thus chloroquine hinders the entry of viruses into the host cell via this mechanism. However, the effects on viral glycosylation have been hypothesized to be not a direct consequence of it's effects on quinone reductase 2 but, rather, on the possible effects of the drug on UDP-N-acetylglucosamine 2-epimerases, which are structurally related but distinct enzymes. If the drug is provided early enough, (hydroxy)chloroquine may have an impact on the magnitude of the viral load peak/viral clearance (i.e., when the virus is still confined within the pharyngeal cavity). This mechanism has however been derived from bioinformatic results and is still hypothetical at present. Chloroquine/ hydroxychloroquine's effect can only be completely understood when taking into account its ability to raise the virucidal effect on SARS CoV2-infected cells, in this case through boosting the cell-mediated immune response.
Another merit of chloroquine includes its activity to alter iron metabolism, affecting its homeostasis at several stages (Roldan et al., 2020). It suppresses the iron release from transferrin incorporated in endosomes. Iron is essential for the production of deoxyribonucleotides, through the iron-dependent enzyme deoxyribonucleotide reductase. Chloroquine/hydroxychloroquine inhibits lymphocyte proliferation by engaging in this action. It also induces cellular iron starvation which is considered as a possible inhibition of SARS COV2 and useful modulation of immune responses. Hormone hepcidin is increased during infection and inflammation, chloroquine reduces this hormone release and inflammatory cytokines, thus help in recovering anemia and thrombosis (Roldan et al., 2020).
Chloroquine and hydroxychloroquine are powerful ‘anti inflammatory’ drugs and ‘immunomodulators’. These were broadly used for decades as a remedial measure for ‘malaria’ and ‘autoimmune diseases’ inclusive of ‘rheumatoid arthritis’, ‘systemic lupus erythematosus’, etc. They can lower the production of proinflammatory cytokines, such as ‘tumor necrosis factor α (TNFα)’, interleukins ‘IL-1, IL-6 and, interferon-γ’ (Jang et al., 2006; Picot et al., 1991; Van den Borne et al., 1997; Sperber et al., 1993). Moreover, they inhibit the innate immune response by (i) blocking the interaction of cytosolic DNA with the nucleic acid sensor cGAMP synthase (Zhang et al., 2020), (ii) interaction of ‘toll-like receptors’ with ‘nucleic acid ligands’ (Hong et al., 2004; Zhu et al., 2012; Yasuda et al., 2008). In severe COVID-19 infection, the hyperactive immune response is responsible for pneumonia. Hydroxychloroquine is also a lysosomotropic inhibitor, an interferon blocker, which decreases the TNFα release, and suppresses TNF receptors of monocytes, thus reducing the inflammatory immune response to viral infection.
3 Adverse side effects of (hydroxy)chloroquine
The most widely recognized unsafe impacts of (hydroxy) chloroquine are gastrointestinal side effects, like nausea, vomiting, and stomach distress, unusually hepatotoxicity, blindness/visual complications, toxic epidermal necrolysis, cardiotoxicity; and, very rare urticaria, ototoxicity, and neurological symptoms (Munster et al., 2002). The incidence of visual complications is typically uncommon. It’s binding to melanin can lead to ocular pigmentation, leading to visualization-related complications (Yam and Kwok, 2006; Michaelides et al., 2011; Wolfe and Marmor, 2010). Proximal myopathy related to respiratory failure in older patients receiving any of these drugs due to ceaseless rheumatoid arthritis or immune system disorders has also been reported (Siddiqui et al., 2007; Kwon et al., 2010; Abdel-Hamid et al., 2008; Becerra-Cunat et al., 2003). (Hydroxy) chloroquine-associated cardiac disease is an exceptional but may cause severe adverse effect resulting in death (Chatre et al., 2018). Human-ether-a-go-go-related gene (hERG) dysfunction causes chronic QT syndrome and sudden death, which occurs in patients with cardiac ischemia. Although small doses of (hydroxy)chloroquine are generally safe, both of which can block the hERG channel (Giudicessi et al., 2020; Naksuk et al., 2020). Adversity is dose-dependent and may vary from person to person. It has been reported that “an amount of 600 mg increased the mean ‘QTc’ by 6.1 ms, whereas a 1200 mg dose led to the increase of the mean QTc by 28 ms” (Pukrittayakamee et al., 2014; Mzayek et al., 2007; Zhang et al., 2020). Long-term use of (Hydroxy)chloroquine has been reported to cause prolonged QT intervals and severe arrhythmia (Chen et al., 2006; Stas et al., 2008). These drugs are reported to block the Ik current, slow down the rate of deactivation and increase the transport of hERG protein. In addition, if (hydroxy)chloroquine is combined with CYP3A4 inhibitors like anti-flu medicines like lopinavir/ritonavir/azithromycin, the risk of QT prolongation may increase and could cause serious arrhythmias by blocking or inhibiting cardiac potassium channels (Zhang et al., 2020; Zequn et al., 2021; Wu et al., 2020). The possible mechanism of SARS COV2 infection causing pathologies in multiple organs is shown in Fig. 1.Possible mechanism of SARS COV2 infection causing pathologies in multiple organs (Violet arrows). Black dotted arrows show the (hydroxy) chloroquine therapeutic action to compete with receptors on different organs of SARS COV2 affected individuals and potential toxic effects. The left side of the figure shows its effect on the hERG channel inducing prolonged QT interval and cardiac arrhythmia.
4 Zinc oxide nanoparticles
Due to the toxic side effects of (hydroxy)chloroquine or their combinations with anti-flu medicines, there is a pressing need for new, and safe chemotherapeutic agents. The development of potential therapeutics requires understanding the entry of the virus into the cells, a key element in viral infection. Various researchers have elucidated insights into the endocytic path and the autophagy route in viral entrance and viral multiplication. As an outcome, the endosomes and lysosomes are considered significant targets for developing therapeutic strategies to combat diseases caused by CoVs.
Cellular enzymes and transcription factors use zinc ions to play a crucial role in various activities. Intracellular zinc concentrations can constrain RNA-dependent RNA polymerases and other proteins critical for completing various viral life cycle stages. Zinc has an intrinsic antiviral property, as mentioned above in the article. Zinc deficiency is responsible for 16 % of all deep respiration infections globally (Wessels et al., 2020; World Health Organization, 2002) suggesting the association of zinc deficiency with the threat of infection and excessive development of COVID-19. Zinc supplementation improves mucociliary clearance (Darma et al., 2020) strengthens epithelial integrity (Roscioli et al., 2017) reduces viral replication (Hamdi et al., 2021), and maintains antiviral immunity (Razzaque, 2021; Maares and Haase, 2016). As a result, it reduces lung damage and secondary infections (Wessels et al., 2020; Razzaque, 2021). Given the well-established therapeutic use of ZnO against herpes simplex and influenza viruses (Tavakoli et al., 2018; Ghaffari et al., 2019), exploiting ZnO NPs against the virus would be a potential approach, due to the unique and distinctive characteristics of nanoparticles as compared to conventional materials. ZnO NPs possess attractive optical, piezoelectric, magnetic, and sensing characteristics. Due to nano size, their outer surfaces can be tailored to present cationic, anionic, polar, nonpolar, or neutral faces to the nearby milieu (Kim, 2007). ZnO NPs interact electro-statically with viral-like proteins using a core–shell model approach (Phan and Hoang, 2019). ZnO NPs bind with DNA/RNA, preferably with the ring nitrogen atom or top position of the nucleobases. A recent report regarding the inactivation of the H1N1 influenza virus by ZnO NPs (Ghaffari et al., 2019) is influential and suggests exploiting its antiviral activity against coronaviruses. In silico molecular docking suggested the possible interactions between ZnO NPs and COVID-19 targets, including ACE 2 receptors, RNA-dependent RNA polymerase, and COVID-19 protease (Hamdi et al., 2021). The most likely mechanism includes a potential attachment with viral virions (spike proteins) and blocking the host receptors ACE 2 for interaction, internalization of ZnO NPs inhibit early viral replication cycle followed by the release of zinc ions which disturbs the plasmid/viral integrity; lastly, reactive oxygen species are produced photo catalytically to potentially deprive lipid/ protein, and nucleic structure of SARS CoV2. At very low concentrations (10 µM), zinc ions can inhibit ACE 2′s capacity to metabolize substrates to provide antiviral effects (Sportelli et al., 2022).
Zinc ionophores augment the intracellular zinc concentration by facilitating the zinc ions into the cells and subsequently impairing virus replication. Chloroquine phosphate acts as a zinc ionophore and it directs zinc to lysosomes (Xue et al., 2014). Both zinc and chloroquine are FDA-approved and readily available. In another molecular docking investigation, the binding site interaction showed that the communication between ‘Zn (Chloroquine) Cl2 (H2O)’ and the ‘protease’ of SARS CoV2 showed 3 hydrogen bonds, while the ‘Zn (hydroxychloroquine)Cl2 (H2O)’ depicted the solid binding to ‘protease’ receptors due to 8 hydrogen bond formation (Hussein and Elkhair, 2021), which is suggestive of the strong binding of hydroxychloroquine as compared to chloroquine. Chloroquine is water soluble and has two basic groups that correspond to the “quinoline-ring nitrogen” and the “diethylamino side-chain nitrogen,” with an ionization constant’ of 8.1 and 10.2′, respectively. The ability of chloroquine to traverse biological membranes and accumulate in acidic organelles is connected to both its acid-base characteristics and the protonation state of nitrogen atoms, which are closely tied to the chloroquine coordination (Paulikat et al., 2022). At 7.2–7.4 (physiological pH), chloroquine binds to Zn2+ via the ‘quinoline-ring nitrogen’ in a tetrahedral complex forming the coordination sphere to produce either a ‘zwitterionic’ complex which is stable at pH 7 (neutral) or ‘cationic’ complex. The metal coordination is lost at somewhat low pH below 6, suggesting that Zn2+ ions are released into the lysosomal lumen. Fig. 2 depicts the schematic illustration of binding of zinc ions with quinoline nitrogen of chloroquine to form chloroquine-zinc complex. Eighteen percent of chloroquine is monoprotonated at physiologic pH, but it is still lipid soluble and may penetrate cell membranes. Chloroquine that has been biprotonated and is present in lysosomes at pH 4–5 is sequestered and prohibited from returning to the cytoplasm. Although the quantity of free chloroquine in the blood is negligible at physiologic pH, this form of the drug determines its distribution between body tissues and blood.Schematic illustration of binding of zinc ions with quinoline nitrogen of chloroquine to form chloroquine-zinc complex.
Zinc also exhibits anti-inflammatory properties barring NF-kB signaling and regulatory T-cell roles that may limit the cytokine storm. Zinc ions interact directly with the hERG channel, and the interaction leads to an adjustment of the channel deactivation mechanism (Anumonwo et al., 1999; Piscopo and Brown, 2018). It has been reported that it significantly reduces the rate of hERG current inactivity during the stabilization, accelerates the closure of the channel during renewable tails, and shows significant fluctuations in current performance or power dependencies.
It is tough to obtain dispersible ZnO or ZnO NPs in aqueous solutions. In our work, we developed a novel method for preparing dispersible suspensions of ZnO NPs possessing flowerlike morphology with good dispersion and high yield in a short period (Manuja et al., 2020). The dispersed ZnO NPs may penetrate easily through the host cell membrane to combat the virus particles.
Although nanoparticles hold novel properties that can enhance their efficacy, but they can be toxic when they get in touch with biological systems. Numerous unique characteristics of NPs, including their size, shape, charge, crystal structure, surface area, sensitivity to certain cell types, and other characteristics, impact both the toxicity of NPs as well as their mode of action in biological applications (Manuja et al., 2021). For instance, DNA has a diameter of 2 nm, but the typical cell membrane thickness is approximately10 nm; as a result, the particle size will aid with the possible internalization of NP within a cell. Similarly, the surface charge drives a predictable aspect of NP uptake in cells. Cationic NPs stimulate endocytosis by expressing affinity for anionic phospholipid membranes. When exposed to a biological environment, ZnO NPs have a tendency to scatter and release ions, which causes the generation of reactive oxygen species (ROS) and oxidative stress (Raguvaran et al., 2017). ZnO NPs' toxicity has been thoroughly investigated and has been proven to have an impact on a variety of cell types and animal systems. We have already reported the toxicity of ZnO NPs (Raguvaran et al., 2017; Raguvaran et al., 2015) and the resolving through polymeric delivery in our previous work (Raguvaran et al., 2017; Chopra Meenu et al., n.d.; Raguvaran et al., 2017; Manuja et al., 2020). To address the toxicity issues, the proper delivery of ZnO NPs along with (hydroxy)chloroquine (as an ionophore and antiviral property) may pave the way for more efficient therapy.
5 Delivery options
Carriers/nanocarriers play a vital role in drug delivery to overcome the toxic effects. The primary benefit of the delivery vehicle is reducing the adverse effects and improving therapeutic efficacy at low concentrations. It should have proven characteristics of enhancing the drug bioavailability and prolonging the duration in blood circulation with sustained release and targeting ability. Liver, spleen, bone marrow, and lung tissues serve as the main route of elimination. Large numbers of phagocytic cells (such as macrophages), which identify nanoparticles as foreign objects and effectively remove them from the bloodstream, are present in these tissues. The opsonization of the nanoparticle by serum proteins, such as immunoglobulins and complement proteins, which results in more effective phagocyte recognition, can increase the pathway's effectiveness. Contrarily, by making nanoparticles more “stealthy” through methods like PEG conjugation, this clearance mechanism can be delayed (Klibanov et al., 1990). The PEGylation can alter the hydrophilicity, diameter, and shape of nanoparticles, among other physicochemical characteristics.
Nanomaterials used for delivery have been shown to lower the toxicity of antiviral agents (Lembo and Cavalli, 2010; Cojocaru et al., 2020). Since (hydroxy) chloroquine is a zinc ionophore besides an antiviral agent. It is pertinent to focus on Zn/chloroquine's delivery applications like (i) copolymer micelles, (ii) pH-sensitive delivery, and (iii) encapsulation of the drugs or molecules or their combinations. We have demonstrated that when metal nanoparticles are added to a polymer hydrogel matrix, their toxicity is reduced and their efficacy is improved due to sustained and controlled release (Raguvaran et al., 2017; Chopra Meenu et al., n.d.; Raguvaran et al., 2017; Manuja et al., 2020).
-
Copolymer micelles
Copolymer micelles are quickly becoming dominant platforms for drug delivery applications because of their small size, ability to solubilize water-insoluble drugs, and extended blood circulation. The application or potential application of ZnO NPs in therapy and vaccine to fight COVID-19 has been discussed (Faizan, 2021; Croy and Kwon, 2006). ZnO NPs are mentioned as antiviral, easy to prepare economically but toxic, whereas polymer micelles preparation is comparatively complex, costly. Polymeric micelles are more biocompatible, soluble, elicit high immune response as compared to ZnO NPs. Table 1 summarized the comparison between ZnO NPs and polymer/micelles used in therapy or used in the vaccine to fight COVID-19 impact (Supplementary file).
ZnO NPs
POLYMER Micelles
Antiviral
yes
–
Preparation
Easy
Complex
Cost
Economical
Costly
Solubility
Less
More
Biocompatibility
Less
High
Immune response
Comparatively less immune response.
strong cellular Immune response.
Cytokines
Minimum secretion of cytokines
Increased secretion of cytokines
Antigen Antibody Interaction.
Decreased levels of antibodies and antigen specific antibodies.
Increased levels of antibodies and antigen-specific antibodies (i.e., IgA, IgG, etc.)
Adjuvant properties
Adjuvant properties are not much advanced
Having advanced adjuvant properties
Dosage formulations
More than single dose formulations
single dose formulations
Toxicity
Toxic
Comparatively safe
The drawbacks of polymeric micelles include early and insufficient release into the diseased tissue (Miller et al., 2013). However, stabilization of the suitable micelle or strong drug interaction through hydrogen/covalent bonding can solve this problem.
PEGylation is a pertinent process in which polyethylene glycol (PEG) is combined with another molecule with promising therapeutic properties. PEG with good bio-compatibility and hydrophilic formation of core in micelles can reduce the nonspecific adhesion to different components of the bloodstream and prolong the duration of its blood circulation. The chloroquine incorporated Zn particles and PEG/PLA may be linked by various interactions such as Hydrophobic-hydrophobic, Ion-dipole, H-bond, and Dipole-dipole as shown in Fig. 3A. Among all interactions, solute-hydrophobic and hydrophobic-hydrophobic interactions are dominant over solute-hydrophilic and hydrophilic-hydrophobic interactions (Masood et al., 2020). Hydrophobic (e.g., PLA, etc.) and hydrophilic (PEG) polymers/entities create the hydrophobic core and the hydrophilic covering shell parts, respectively, of the copolymer micelles (Fig. 3B). The drug is sheltered from enzymatic degradation by the micelle's shell; it remains in the blood for a prolonged period. The micelles' surface properties, size, and stability primarily determine their biodistribution. They traverse the fenestrated blood vessels/capillaries that arise in most tissues (Hill et al., 2012). The inflamed tissues usually have permeable blood vessels/capillaries with big fenestra, so the permeation of micellar drug complexes into such tissues is quicker than into healthy tissues because of passive targeting and selective distribution to the diseased site. One can achieve specific targeting by attaching it to the human monoclonal or polyclonal antibodies directed against spike proteins of the SARS COV2, thus preventing the virus from latching onto the other cells.
-
pH responsive micelles
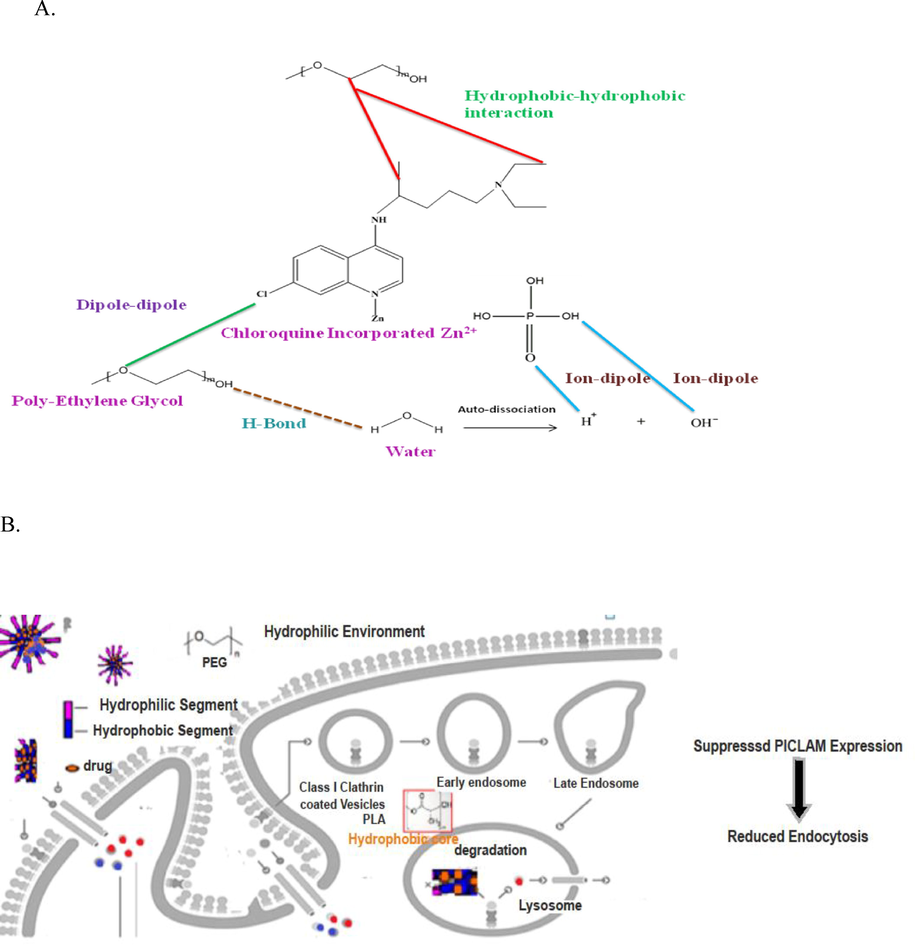
- A. Possible interactions between chloroquine incorporated Zn particles and PEG/PLA. Hydrophobic-hydrophobic, Ion-dipole, H-bond, and Dipole-dipole are shown by red, blue, dotted brown and green colors respectively. B. Schematic illustration of intracellular delivery of (hydroxy)chloroquine and zinc through copolymer micelles. The micelles contain hydrophilic and hydrophobic polymer entities. The drug may suppress the expression of phosphatidyl inositol binding clathrin assembly protein (PICALM) and reduce the pace of endocytosis in the hydrophobic core.
Smart block copolymers, which are responsive to pH, temperature, ultrasound, or light, can allow controlled dissociation of the micelle and well-organized drug release. The pH-responsive micelles can be designed and synthesized for target delivery, exploiting the pH-dependent interaction of the virus and cell membrane. For various biological applications, pH-responsive systems ought to be reactive and steady to somewhat lower (5.0–6.5 pH), and 7.4 pH (physiological pH). The pH-sensitive formulation can be fabricated to deliver the zinc/(hydroxy)chloroquine with or without another antiviral agent allowing the release in an acidic milieu. This design can help the Zn/chloroquine liberation within the cell and adjacent tissues. It is due to the endo lysosomal sections formed upon the internalization of cargo. Micelles will be stable at neutral pH but allow the fast drug release in endocytic pH. Positively charged micelles can be safeguarded by the negatively charged entities at pH 7.4 and they can be broken or unprotected at the diseased sites’ pH due to pH-responsive entity. The pH-sensitive linkages responsive to low pH (endosomal pH) can be utilized in the design. Following endocytosis, the cationic entity will be protonated in the endosomal area resulting in the disintegration of the micelle and destabilizing the endosomal membrane and thus supporting the delivery of the zinc/(hydroxy)chloroquine to the cytosol.
Although some pH-sensitive nanoparticles have good in vitro reactivity or activity, they may be hampered in vivo by a complicated physiological or pathological milieu. The low levels of the drug/molecule may inhibit the response activity of a given pH-sensitive component. The breakage of pH-sensitive chemical bonds may take longer, affecting the release of drug/molecule from the delivery system (Mu et al., 2021). A more sensitive and particular pH should be required with a subtle polymer design (Zhuo et al., 2020). A well-built library of materials with varied pH conversions to get suitable polymers can be created (Ma et al., 2014).
-
Encapsulation
The exploitation of natural polymers is valuable based on established biocompatibility. Chitosan can be used as a functional carrier for zinc and chloroquine, reducing both components' adverse effects. The delivery materials could also function as camouflage to deter immune responses or as promoters that could propel or respond to specific molecules or chemical processes. Chitosan is a customized biopolymer accomplished by de-acetylation of natural chitin, comprising N-acetyl glucosamine and glucosamine units (Rashki et al., 2020). It is a cationic polysaccharide polymer that can bind easily with the drug and is biocompatible and biodegradable. Additionally, it enhances the transport of drugs across the cell membrane. It can suppress multiple efflux pumps, thereby avoiding the problem of drug resistance (Ngo et al., 2015). The quick efflux lowers the intracellular drug concentration (Boroumand et al., 2021). The metabolism of chloroquine in the liver and small intestine, which produces quick clearance, is another explanation put forth for its decreased bioavailability. Higher dosages must be used because of the medicine's low bioavailability, which may also promote the development of drug resistance. The use of degradable polymers like chitosan as a carrier may protect the drug and avoid its renal clearance. The chitosan itself will be metabolized and degraded by enzymes in the body, eventually being removed by renal clearance. Several researchers employ chitosan as a delivery vehicle for antiviral drugs such as saquinavir, a protein inhibitor affecting viral proliferation of HIV (Ramana et al., 2014), Acyclovir against Herpes simplex virus-1 (Donalisio et al., 2018), and influenza vaccine (Dehghan et al., 2014) with greater efficacy as compared to their counterparts.
Chitosan has a remarkable water absorption capacity and it swells as a hydrogel. At physiologic/basic pH, it becomes insoluble. However, it may dissolve in acidic conditions. This property can be exploited for the release of chloroquine/hydroxychloroquine to the affected areas with an acidic environment. It was noted that the hydrogel showed higher drug release at pH 5.7 than at pH 7.4. The chemical and graphical illustration of encapsulation of chloroquine-zinc complex and decapsulation at different pH of the body is shown in Fig. 4 A and B respectively. At physiologic/basic pH, it becomes insoluble. It dissolves in acidic conditions. Given the acidic milieu of endosomes (pH 4.5–6.0) and lysosomes (pH 4.5–4.8) compared to a body pH of 7.4, chitosan-based stimulant-sensitive hydrogels can be synthesized and developed for enhanced drug release in the endosomal or lysosomal compartments using internal pH stimuli (Chen et al., 2009; Du et al., 2005).Chemical (A) and Graphic illustration (B) of encapsulation of chloroquine-zinc complex and decapsulation at different pH of the body. At physiologic/basic pH, it becomes insoluble. It dissolves in acidic conditions. The chitosan hydrogel showed higher drug release at pH 5.7.
As referred to earlier reports, chitosan carriers are becoming increasingly popular because of their numerous benefits. However, this polysaccharide still faces several limitations, such as the low solubility at blood pH, premature release, and its structural instability after cellular uptake. Therefore, it is obligatory to find some improvement in chitosan-related nanocarriers. In a study, chitosan integrated albumin was observed as a perfect carrier for muco-inhalable delivery of silymarin/curcumin against SARS COV2 (Hanafy and El-Kemary, 2022). Chitosan can alter the physiochemical characteristics of nanoparticles, increasing their dispersibility and bioavailability in the lungs. Researchers used the chemical alteration of chitosan using PEG (PEGylation) to improve the melting of chitosan, even though extreme PEGylation may reduce its solubility, charge, and binding capacity to DNA/RNA (Boroumand et al., 2021).
Despite all of the progress in understanding ZnO nanostructures' mechanisms of action and biological impacts, there is still a paucity of knowledge regarding the long-term implications. As a result, further information is needed to determine if the numerous proven benefits of ZnO outweigh the potential hazards.
In summary, the toxicity issues or adverse effects relating to the decades-old known drug (hydroxy)chloroquine are associated with its improper use concerning dose and its interaction with other medicines provided to COVID-19 patients. Chloroquine is a well-known zinc ionophore besides possessing an antiviral effect. Smart and targeted delivery, including nanotechnology approaches to deliver ZnO/hydroxychloroquine, can be exploited. It will enable us to deliver inaccessible drugs with diminished toxicity, improved solubility, controlled/ sustained release and site-specific delivery through pH-sensitive copolymer micelles/drug encapsulation. One of the most difficult obstacles has been the low pH range, which requires the micellar form to hold the medicine for a longer duration before releasing it. Because of the moderate acidic environment and dynamic distribution of nanoparticles in vivo, a quick response time is required for precise drug release. We discussed the delivery options like copolymer micelle/pH-sensitive/encapsulation of ZnO NPs along with (hydroxy) chloroquine (as an ionophore) which would be the better option for therapeutic management of COVID-19. The use of FDA-approved materials for the delivery or various therapies has favorable risk: benefit profiles and may shorten the development and approval procedures.
6 Authors’ contributions
Anju Manuja and Balvinder Kumar conceived the idea; Anju Manuja wrote the article, Dharvi Chhabra collected the matter and assisted in figures. Balvinder Kumar edited the manuscript.
Acknowledgment
Authors thank Director, ICAR-National Research Centre on Equines, Hisar, Haryana, India for providing administrative support and Ms Swati Rani for drawing the chemical structures.
Declaration of Competing Interest
The authors declare that they have no known competing financial interests or personal relationships that could have appeared to influence the work reported in this paper.
References
- Severe hydroxychloroquine myopathy. Muscle Nerve: Off. J. Am. Assoc. Electrodiag. Med.. 2008 Sep;38(3):1206-1210.
- [Google Scholar]
- Chloroquine could be used for the treatment of filoviral infections and other viral infections that emerge or emerged from viruses requiring an acidic pH for infectivity. Cell Biochem. Funct.. 2016 Jun;34(4):191-196.
- [Google Scholar]
- Targeting endosomal acidification by chloroquine analogs as a promising strategy for the treatment of emerging viral diseases. Pharmacol. Res. Perspect.. 2017 Feb;5(1):e00293.
- [Google Scholar]
- Bansal et al., 2021. Hydroxychloroquine: a comprehensive review and its controversial role in coronavirus disease, Annals of Medicine, 53:1, 117-134
- Chloroquine-induced myopathy and neuropathy: progressive tetraparesis with areflexia that simulates a polyradiculoneuropathy. Two Case Rep. Rev. Neurol.. 2003 Mar 1;36(6):523-526.
- [Google Scholar]
- Amodiaquine, an antimalarial drug, inhibits dengue virus type 2 replication and infectivity. Antivir. Res.. 2014;106:125-134.
- [Google Scholar]
- Chitosan-based nanoparticles against viral infections. Front. Cell. Infect. Microbiol.. 2021 Mar;17(11):175.
- [Google Scholar]
- Cardiac complications attributed to chloroquine and hydroxychloroquine: a systematic review of the literature. Drug Saf.. 2018 Oct;41(10):919-931.
- [Google Scholar]
- pH-responsive biodegradable micelles based on acid-labile polycarbonate hydrophobe: synthesis and triggered drug release. Biomacromolecules. 2009 Jul 13;10(7):1727-1735.
- [Google Scholar]
- Chronic hydroxychloroquine use associated with QT prolongation and refractory ventricular arrhythmia. Clin. Toxicol.. 2006 Jan 1;44(2):173-175.
- [Google Scholar]
- Chopra Meenu, Bernela Manju, Kaur Pawan, Manuja Anju, Kumar Balvinder, Thakur Rajesh Alginate/gum acacia bipolymeric nanohydrogels—Promising carrier for Zinc oxide nanoparticles.International Journal of biological macromolecules.72c:827-833.
- Nanomaterials designed for antiviral drug delivery transport across biological barriers. Pharmaceutics. 2020 Feb;12(2):171.
- [Google Scholar]
- Zinc supplementation effect on the bronchial cilia length, the number of cilia, and the number of intact bronchial cell in zinc deficiency rats. Indonesian Biomed. J.. 2020;12(1):78-84.
- [Google Scholar]
- Rabbit nasal immunization against influenza by dry-powder form of chitosan nanospheres encapsulated with influenza whole virus and adjuvants. Int. J. Pharm.. 2014 Nov 20;475(1–2):1-8.
- [Google Scholar]
- Chloroquine, an endocytosis blocking agent, inhibits Zika virus infection in different cell models. Viruses. 2016;8(12):322.
- [Google Scholar]
- Acyclovir-loaded chitosan nanospheres from nano-emulsion templating for the topical treatment of herpesviruses infections. Pharmaceutics. 2018 Jun;10(2):46.
- [Google Scholar]
- pH-sensitive vesicles based on a biocompatible zwitterionic di block copolymer. J. Am. Chem. Soc.. 2005 Dec 28;127(51):17982-17983.
- [Google Scholar]
- Zinc oxide nanoparticles to fight the COVID-19. Acta Scient. Agric.. 2021;5(7):14-16.
- [Google Scholar]
- Chloroquine inhibits dengue virus type 2 replication in Vero cells but not in C6/36 cells. Sci. World J. 2013282734
- [Google Scholar]
- Breakthrough: chloroquine phosphate has shown apparent efficacy in treatment of COVID19 associated pneumonia in clinical studies. Biosci. Trends 2020
- [Google Scholar]
- Hydroxychloroquine and azithromycin as a treatment of COVID19: results of an open-label non-randomized clinical trial. Int. J. Antimicrob. Agents. 2020 Jul 1;56(1):105949
- [Google Scholar]
- Inhibition of H1N1 influenza virus infection by zinc oxide nanoparticles: another emerging application of nanomedicine. J. Biomed. Sci.. 2019;26(1):1.
- [Google Scholar]
- Giudicessi JR, Noseworthy PA, Friedman PA, Ackerman MJ. Urgent guidance for navigating and circumventing the QTc-prolonging and torsadogenic potential of possible pharmacotherapies for coronavirus disease 19 (COVID19). In Mayo Clinic Proceedings 2020 Jun 1 (Vol. 95, No. 6, pp. 1213-1221). Elsevier.
- Investigating the internalization and COVID19 antiviral computational analysis of optimized nanoscale zinc oxide. ACS Omega. 2021;6(10):6848-6860.
- [Google Scholar]
- Silymarin/curcumin loaded albumin nanoparticles coated by chitosan as muco-inhalable delivery system observing anti-inflammatory and anti COVID-19 characterizations in oleic acid triggered lung injury and in vitro COVID-19 experiment. Int. J. Biol. Macromol.. 2022;198:101-110.
- [Google Scholar]
- Hill RG, Richards D, editors. Drug Discovery and Development E-Book: Technology in Transition. Elsevier Health Sciences; 2012
- SARS-CoV-2 cell entry depends on ACE2 and TMPRSS2 and is blocked by a clinically proven protease inhibitor. Cell 2020 Mar 5
- [Google Scholar]
- Hong Z, Jiang Z, Liangxi W, Guofu D, Ping L, Yongling L, Wendong P, Minghai W. Chloroquine protects mice from challenge with CpG ODN and LPS by decreasing proinflammatory cytokine release. International immunopharmacology. 2004 Feb1;4(2):223
- http.//Clinical trials.gov.in as assessed on date 11/11/2022.
- Insights from nanomedicine into chloroquine efficacy against COVID19. Nat. Nanotechnol. 2020:1-3.
- [Google Scholar]
- Diabetes mellitus is associated with increased mortality and severity of disease in COVID19 pneumonia–a systematic review, meta-analysis, and meta-regression. Diabetes Metab. Syndr.. 2020 Jul 1;14(4):395-403.
- [Google Scholar]
- Molecular docking identification for the efficacy of some zinc complexes with chloroquine and hydroxychloroquine against main protease of COVID19. J. Mol. Struct.. 2021;1231:129979
- [Google Scholar]
- Chloroquine inhibits production of TNF-α, IL-1β and IL-6 from lipopolysaccharide-stimulated human monocytes/macrophages by different modes. Rheumatology. 2006 Jun 1;45(6):703-710.
- [Google Scholar]
- Expert consensus on chloroquine phosphate for the treatment of novel coronavirus pneumonia. Zhonghua Jie He He Hu Xi Za Zhi. 2020;43(3):185-188.
- [Google Scholar]
- Lysosomal sequestration of amine-containing drugs: analysis and therapeutic implications. J. Pharm. Sci.. 2007 Apr 1;96(4):729-746.
- [Google Scholar]
- Kim KY. Nanotechnology platforms and physiological challenges for cancer therapeutics. Nanomedicine: Nanotechnology, Biology and Medicine. 2007 Jun 1;3(2):103-10.
- Amphipathic polyethyleneglycols effectively prolong the circulation time of liposomes. FEBS Lett.. 1990;268(1):235-237.
- [Google Scholar]
- Kinetic mechanism of quinone oxidoreductase 2 and its inhibition by the antimalarial quinolines. Biochemistry. 2004;43:4538-4547.
- [Google Scholar]
- Hydroxychloroquine-induced myopathy. JCR J. Clin. Rheumatol.. 2010 Jan 1;16(1):28-31.
- [Google Scholar]
- Lancet, T., Expression of concern: Hydroxychloroquine or chloroquine with or without a macrolide for treatment of COVID-19: a multinational registry analysis. Lancet (London, England).
- Nanoparticulate delivery systems for antiviral drugs. Antivir. Chem. Chemother.. 2010 Dec;21(2):53-70.
- [Google Scholar]
- Liu J, Cao R, Xu M. Hydroxychloroquine, a less toxic derivative of chloroquine, is effective in inhibiting SARS-CoV-2 infection in vitro [published online March 18, 2020]. Cell Discovery.
- Ultra-pH-sensitive nanoprobe library with broad pH tunability and fluorescence emissions. J. Am. Chem. Soc.. 2014 Aug 6;136(31):11085-11092.
- [Google Scholar]
- Zinc and immunity: an essential interrelation. Arch. Biochem. Biophys.. 2016 Dec;1(611):58-65.
- [Google Scholar]
- Microwave assisted fast fabrication of zinc/iron oxides based polymeric nanocomposites and evaluation on equine fibroblasts. Int. J. Biol. Macromol.. 2020;165:71-81.
- [Google Scholar]
- Accelerated healing of full thickness excised skin wound in rabbits using single application of alginate/acacia based nanocomposites of ZnO nanoparticles. Int. J. Biol. Macromol. 2020 Mar 28
- [Google Scholar]
- Metal/metal oxide nanoparticles: toxicity concerns associated with their physical state and remediation for biomedical applications. Toxicol. Rep.. 2021;8:1970-1978.
- [Google Scholar]
- Drug-drug and drug-solvent interaction studies of Chloroquine phosphate, Acefylline piperazine and Gentamicin sulfate in polymeric systems. Arab. J. Chem.. 2020;13(7):6221-6235.
- [Google Scholar]
- Coronavirus disease (COVID-19): comprehensive review of clinical presentation. Front. Public Health. 2021 Jan;15(8):1034.
- [Google Scholar]
- Retinal toxicity associated with hydroxychloroquine and chloroquine: risk factors, screening, and progression despite cessation of therapy. Arch. Ophthalmol.. 2011 Jan 10;129(1):30-39.
- [Google Scholar]
- Premature drug release of polymeric micelles and its effects on tumor targeting. Int. J. Pharm.. 2013 Mar 10;445(1–2):117-124.
- [Google Scholar]
- Hydroxychloroquine concentration–response relationships in patients with rheumatoid arthritis. Arthrit. Rheumat.: Off. J. Am. College Rheumatol.. 2002 Jun;46(6):1460-1469.
- [Google Scholar]
- Randomized dose-ranging controlled trial of AQ-13, a candidate antimalarial, and chloroquine in healthy volunteers. PLoS Clin. Trials. 2007 Jan 5;2(1):e6.
- [Google Scholar]
- Cardiac safety of off-label COVID19 drug therapy: a review and proposed monitoring protocol. Eur. Heart J. Acute Cardiovasc. Care. 2020 Apr;9(3):215-221.
- [Google Scholar]
- Biological effects of chitosan and its derivatives. Food Hydrocoll.. 2015 Oct 1;51:200-216.
- [Google Scholar]
- In vitro inhibition of human influenza A virus replication by chloroquine. Virol. J.. 2006;3:39.
- [Google Scholar]
- Hydroxychloroquine-mediated inhibition of SARS-CoV-2 entry is attenuated by TMPRSS2. PLoS Pathog.. 2021;17(1):e1009212.
- [Google Scholar]
- Paulikat, M., Vitone, D., Schackert, F.K., Schuth, N., Barbanente, A., Piccini, G., Ippoliti, E., Rossetti, G., Clark, A.H., Nachtegaal, M. and Haumann, M., 2022. Molecular dynamics and structural studies of zinc chloroquine complexes.
- Phan AD, Hoang TX. The pH-dependent electrostatic interaction of a metal nanoparticle with the MS2 virus-like particles. Chemical Physics Letters. 2019 Sep1;730:84-8
- Picot S, Peyron F, Vuillez JP, Polack B, Ambroise-Thomas P. Chloroquine inhibits tumor necrosis factor production by human macrophages in vitro. J Infect Dis. 1991 Oct1;164(4):830
- Piscopo S, Brown ER. Zinc Oxide Nanoparticles and Voltage‐Gated Human Kv11. 1 Potassium Channels Interact through a Novel Mechanism. Small. 2018 Apr;14(15):1703403
- Pharmacokinetic interactions between primaquine and chloroquine. Antimicrob. Agents Chemother.. 2014 Jun 5;8(6):3354-3359.
- [Google Scholar]
- Zinc oxide nanoparticles induced haemolytic cytotoxicity in horse red blood cells. Int. J. Pharm. Sci. Res.. 2015 Mar 1;6(3):1166.
- [Google Scholar]
- Sodium alginate and gum acacia hydrogels of zinc oxide nanoparticles reduce hemolytic and oxidative stress inflicted by zinc oxide nanoparticles on mammalian cells. Int. J. Biol. Macromol.. 2017 Aug;1(101):967-972.
- [Google Scholar]
- Sodium alginate and gum acacia hydrogels of ZnO nanoparticles show wound healing effect on fibroblast cells. Int. J. Biol. Macromol.. 2017 Mar;1(96):185-191.
- [Google Scholar]
- Ramana LN, Sharma S, Sethuraman S, Ranga U, Krishnan UM. Evaluation of chitosan nanoformulations as potent anti-HIV therapeutic systems. Biochimica et Biophysica Acta (BBA)-General Subjects. 2014 Jan 1;1840(1):476-84.
- Rashki S, Asgarpour K, Tarrahimofrad H, Hashemipour M, Ebrahimi MS, Fathizadeh H, Khorshidi A, Khan H, Salavati-Niasari M, Mirzaei H. Chitosan-based nanoparticles against bacterial infections. Carbohydrate Polymers. 2020 Sep 20;117(108)
- Rathore JS, Ghosh C. Severe acute respiratory syndrome coronavirus-2 (SARS-CoV-2), a newly emerged pathogen: an overview. Pathogens and disease. 2020 Aug;78(6):ftaa042
- COVID-19 pandemic: Can zinc supplementation provide an additional shield against the infection? Comput. Struct. Biotechnol. J.. 2021 Jan;1(19):1371-1378.
- [Google Scholar]
- The possible mechanisms of action of 4-aminoquinolines (chloroquine/hydroxychloroquine) against Sars-Cov-2 infection (COVID-19): a role for iron homeostasis? Pharmacol. Res.. 2020 Aug;1(158):104904
- [Google Scholar]
- Zinc deficiency as a codeterminant for airway epithelial barrier dysfunction in an ex vivo model of COPD. Int. J. Chron. Obstruct. Pulmon. Dis.. 2017;12:3503.
- [Google Scholar]
- Hydroxychloroquine-induced toxic myopathy causing respiratory failure. Chest. 2007 Feb 1;131(2):588-590.
- [Google Scholar]
- Sperber K, Quraishi HU, Kalb TH, Panja AS, Stecher V, Mayer L. Selective regulation of cytokine secretion by hydroxychloroquine inhibition of interleukin 1 alpha (IL-1-alpha) and IL-6 in human monocytes and T cells. The Journal of rheumatology.1993 May 1;20(5):803-8.
- On the efficacy of ZnO nanostructures against SARS-CoV-2. Int. J. Mol. Sci.. 2022;23(6):3040.
- [Google Scholar]
- Stas P, Faes D, Noyens P. Conduction disorder and QT prolongation secondary to long-term treatment with chloroquine. International journal of cardiology. 2008 Jul 4;127(2):e80-2.Stas 2008.
- Pharmacokinetic basis of the hydroxychloroquine response in COVID-19: implications for therapy and prevention. Eur. J. Drug Metab. Pharmacokinet.. 2020;45(6):715-723.
- [Google Scholar]
- Polyethylene glycol-coated zinc oxide nanoparticle: an efficient nanoweapon to fight against herpes simplex virus type 1. Nanomedicine. 2018;13(21):2675-2690.
- [Google Scholar]
- Inhibition of human immunodeficiency virus infectivity by chloroquine. AIDS Res. Hum. Retrovir.. 1990;6:481-489.
- [Google Scholar]
- Van den Borne BE, Dijkmans BA, De Rooij HH, Le Cessie S, Verweij CL. Chloroquine and hydroxychloroquine equally affect tumor necrosis factor-alpha, interleukin 6, and interferon-gamma production by peripheral blood mononuclear cells. rheumatology.1997 Jan 1;24(1):55-60.
- Chloroquine is a potent inhibitor of SARS coronavirus infection and spread. Virol. J.. 2005 Dec;2(1):1.
- [Google Scholar]
- Remdesivir and chloroquine effectively inhibit the recently emerged novel coronavirus (2019-nCoV) in vitro. Cell Res.. 2020 Mar;30(3):269-271.
- [Google Scholar]
- Structure of MERS-CoV spike receptor-binding domain complexed with human receptor DPP4. Cell Res.. 2013 Aug;23(8):986-993.
- [Google Scholar]
- Coronavirus pathogenesis and the emerging pathogen severe acute respiratory syndrome coronavirus. Microbiol. Mol. Biol. Rev.. 2005 Dec;69(4):635-664.
- [Google Scholar]
- Efficacy and safety of three new oral antiviral treatment (molnupiravir, fluvoxamine and Paxlovid) for COVID-19: a meta-analysis. Ann. Med.. 2022 Dec 31;54(1):516-523.
- [Google Scholar]
- The potential impact of zinc supplementation on COVID-19 pathogenesis. Front. Immunol. 2020:1712.
- [Google Scholar]
- WHO Solidarity Trial Consortium. Repurposed antiviral drugs for Covid-19—interim WHO solidarity trial results. New England journal of medicine. 2021 Feb 11;384(6):497-511
- Rates and predictors of hydroxychloroquine retinal toxicity in patients with rheumatoid arthritis and systemic lupus erythematosus. Arthritis Care Res.. 2010 Jun;62(6):775-784.
- [Google Scholar]
- World Health Organization. The world health report 2002: reducing risks, promoting healthy life. World Health Organization; 2002
- SARS-CoV-2, COVID19, and inherited arrhythmia syndromes. Heart Rhythm.. 2020 Sep 1;17(9):1456-1462.
- [Google Scholar]
- In vitro antiviral activity and projection of optimized dosing design of hydroxychloroquine for the treatment of severe acute respiratory syndrome coronavirus 2 (SARS-CoV-2) Clin. Infect. Dis.. 2020 Jul 28;71(15):732-739.
- [Google Scholar]
- Yasuda H, Leelahavanichkul A, Tsunoda S, Dear JW, Takahashi Y, Ito S, Hu X, Zhou H, Doi K, Childs R, Klinman DM. Chloroquine and inhibition of Toll-like receptor 9 protect from sepsis-induced acute kidney injury. American Journal of Physiology-Renal Physiology. 2008 May 29; 4(5):1050-8
- Induction of lysosomal dilatation, arrested autophagy, and cell death by chloroquine in cultured ARPE-19 cells. Invest. Ophthalmol. Vis. Sci.. 2010 Nov 1;51(11):6030-6037.
- [Google Scholar]
- Off-label use of chloroquine, hydroxychloroquine, azithromycin and lopinavir/ritonavir in COVID19 risks prolonging the QT interval by targeting the hERG channel. Eur. J. Pharmacol.. 2021 Feb;15(893):173813
- [Google Scholar]
- Pharmacological and cardiovascular perspectives on the treatment of COVID19 with chloroquine derivatives. Acta Pharmacol. Sin.. 2020 Sep;23:1.
- [Google Scholar]
- Targeting Toll-like receptors by chloroquine protects mice from experimental cerebral malaria. Int. Immunopharmacol.. 2012 Aug 1;13(4):392-397.
- [Google Scholar]
- Japanese encephalitis virus enters rat neuroblastoma cells via a pH-dependent, dynamin and caveola-mediated endocytosis pathway. J. Virol.. 2012;86:13407-13422.
- [Google Scholar]