Translate this page into:
Di-block copolymer stabilized methyl methacrylate based polyHIPEs: Influence of hydrophilic and hydrophobic co-monomers on morphology, wettability and thermal properties
⁎Corresponding authors. chm_zhangsx@ujn.edu.cn (Shuxiang Zhang), chm_gengb@ujn.edu.cn (Bing Geng)
-
Received: ,
Accepted: ,
This article was originally published by Elsevier and was migrated to Scientific Scholar after the change of Publisher.
Abstract
Due to intermediate hydrophobicity of methyl methacrylate (MMA) monomer in water, it is difficult to prepare its stable water in oil high internal phase emulsion (HIPE). Moreover, the addition of fully hydrophilic co-monomer such as 2-hydroxyethyl methacrylate (HEMA) in MMA monomer makes it further troublesome to stabilize these emulsions. This paper addresses the preparation of such type of difficult to prepare emulsions via addition of an amphiphilic fluorinated di-block copolymer (FDB), poly(2-dimethylamino)ethylmethacrylate-b-poly(trifluoroethyl methacrylate) (PDMAEMA-b-PTFEMA) as stabilizer. Interestingly, HEMA and/or HFBA (hexa fluorobutyl acrylate) as co-monomers were successfully added to impart some special properties such as thermodynamic stability, desired amphiphilicity to the final polyHIPEs. Fluorinated blocks in FDB anchored well at oil/water interface of HIPE, offering enough hydrophobicity to the comparatively hydrophilic monomers and in turn providing resistance against coalescence. MMA polyHIPEs were found to be fully hydrophobic just by replacing HEMA co-monomer with HFBA. Due to superb inherent hydrophobic nature of fluorine atoms, MMA-HFBA polyHIPEs showed remarkable water contact angle of 139°. Furthermore, the addition of fluorinated co-monomer in MMA based HIPEs significantly improved thermal stabilities of these materials with improvement in degradation temperature from 305 °C to 360 °C.
Keywords
MMA
Porous polymers
Hydrophilic polyHIPE
Hydrophobic polyHIPE
Fluorinated di-block copolymer
RAFT
1 Introduction
High internal phase emulsions (HIPEs) are defined as extremely concentrated emulsions where the dispersed phase occupies more than 74 vol%. PolyHIPEs are interconnected porous polymers that result from polymerizing organic monomers within the continuous phase of HIPEs (Cameron, 2005). PolyHIPEs are important due to wide ranging applications in oil/water separation (Zhang and Guo, 2017), in-situ fire extinguishing (Zhang et al., 2017), tissue engineering (Nalawade et al., 2016), supports for catalysts (Debecker et al., 2015), hazardous metal ions collector (Pan et al., 2016), enhanced gas adsorption/desorption (Wang et al., 2016), dye removal from water (Azhar et al., 2019) and so on. These attractive applications have driven much focus on innovation and development of reliable procedures in the field of HIPE to synthesize porous polymers with desired properties and morphologies. Supercritical carbon dioxide in water (C/W) (C/W) (Mathieu et al., 2018), water in oil (W/O) (Azhar et al., 2017), and oil-in-water (O/W) (Bo et al., 2018) are common types of emulsions used in the synthesis of required polyHIPEs. In literature, Pores (comparatively large windows created by removal of internal droplets in HIPE), and Pore throats (series of small interconnects between pores) are widely used terminologies to explain morphology of polyHIPEs (Stubenrauch et al., 2018).
HIPEs are generally stabilized against coalescence by using large fractions (5–50 wt%) of surfactant molecules (Cameron, 2005; Sun et al., 2010). As these surfactants are toxic in nature and also present in high concentration, hence undesired in practical applications (Wu et al., 2012). It is also noticeable that only a limited number of conventional surfactants are able to stabilize particular monomer/aqueous system of emulsions. Pickering emulsions which are usually particle stabilized emulsions seem a better choice to synthesize polyHIPEs without use of undesirable surfactants. HIPEs stabilized with nanoparticles not only potentially stabilizes emulsions but may also endow a number of benefits in the resulting product which are not possible to achieve when using conventional surfactants, such as, enhancing the surface roughness of polyHIPE and functionalizing the pore walls for use in variety of commercial applications (Zhu et al., 2010). Though Pickering emulsions have enormous benefits compared to surfactant stabilized emulsions, but the problem with these kinds of emulsions is that the resulting polyHIPEs have closed pores (Wenyuan Yi and Wang, 2016). One possible way to combat aforementioned problems is to use surfactant and nanoparticles together in HIPE as stabilizers. By this strategy, interconnected porous structure with the use of least amount of harmful surfactants is possible (Vilchez et al., 2014). Other way is to fabricate novel amphiphilic block copolymers (BCPs) as stabilizers for HIPEs, to wholly decimate the need of harmful conventional surfactants as well as nanoparticles. With the advancement in controlled/living radical polymerization (CLRP) it is now possible to synthesize BCPs with desired molecular weights and narrower polydisperisty with full control. To date, numerous BCPs such as poly(4-vinylpyridine)-b-poly(ethylene glycol)-b-poly(4-vinylpyridine) (Zhang et al., 2016), poly(ethylene oxide)-b-poly(styrene) (Mathieu et al., 2015) and poly(styrene)-b-(acrylic acid) (Luo et al., 2015) among others (Raffa et al., 2015), prepared via CLRP have been reported in order to stabilize HIPEs.
Due to the unique characteristic to tune the porous structure by varying the internal phase fraction, facile preparation and comparatively high stability of HIPEs based on hydrophobic monomers such as styrene (Cameron, 2005; Li et al., 2008); dicyclopentadiene (DCPD) (Kovačič, 2013; Kovačič et al., 2015; Trupej et al., 2017) Glycidyl methacrylate (Yang et al., 2014); caprolactone (Perez-Garcia et al., 2016), and butyl methacrylate (Ma et al., 2014) are abundantly reported in the literature. These hydrophobic monomers are very easy to form W/O HIPEs. It is universally accepted that the monomers containing intermediated hydrophilicities and substantial water solubilities (15 g/L, 25 °C) for instance methyl methacrylate (MMA) are not suitable for preparing stable W/O HIPE due to the immediate phase separation caused by mixing of water phase with oil phase (Cameron, 2005; Li et al., 2016). On the other hand, owing to superb biocompatibility (Vorndran et al., 2012), facile surface modification (David et al., 2000), and high interconnectivity with enhanced mechanical performances as compared to their fully hydrophilic counterparts, MMA polyHIPEs are extremely desirable materials in plenty of applications such as adsorption/ separation, biomaterials (particularly as scaffolds for tissue engineering), to name a few (Serrano-Aroca and Llorens-Gámez, 2017). Until now, only a few research reports on synthesis of MMA polyHIPEs have published due to their considerable high solubility in water and hence difficult to emulsify. Highly mono-dispersed poly(st-co-MMA-co-AA) particles were used to stabilize MMA based Pickering-HIPEs in order to get open porous non-crosslinked foams. Unfortunately, there were only a small amount of interconnected pores compared with those of HIPEs emulsified by common conventional surfactants (Zhang and Chen, 2009). Busby et al. (2001) reported open-cell polyHIPEs containing 20 wt% poly(ɛ-caprolactone) macromonomer and 80 wt% MMA stabilized by blend of SPAN85/PEO-PPO-PEO, however existence of very large closed pores clued the occurrence of HIPE droplet coalescence to a degree. Moreover, blend of surfactants made this system inefficient. Already prepared polyHIPEs from other monomers as oil phase were also used to synthesize a few PMMA containing polyHIPEs (Cummins et al., 2009). A commonly used surfactant span80 was utilized to emulsify MMA HIPEs at room temperature using γ-rays irradiation polymerization by Mao et al. (2013). Although the polyMMA prepared in that work possessed highly open morphology but demand of γ-irradiation facilities limited the scope of that research. In view of all shortcomings associated with the synthesis of MMA based polyHIPEs, an easy and cost efficient synthesis of polyMMA foams with regular and open pores from stable HIPE template is in great demand (Li et al., 2016; Xu et al., 2014).
2-hydroxyethyl methacrylate (HEMA) is a hydrophilic monomer commonly used in biomedical applications such as water uptakes, drug delivery, implants and contact lenses (Kirschner and Anseth, 2013). Based on advantages of HEMA, a few investigations have been conducted to get porous poly(HEMA) via high internal phase emulsion process (Toledo and Urbano, 2016; Kovačič et al., 2007; Majer et al., 2016; Kulygin and Silverstein, 2007). Recently, copolymer of methyl acryl amide (MAA) and HEMA were synthesized to study the effect of concentration of monomers on morphology and swelling performances (Ovadia and Silverstein, 2016). But one problem associated with poly (HEMA) is its poor mechanical performances due to very high swelling in aqueous mediums (Serrano-Aroca and Llorens-Gámez, 2017). On the other hand, partially hydrophilic poly(MMA) has proved to be mechanically robust and also has been approved by US Food and Drug Administration for biomedical applications, hence it is a good tentative idea to use these two monomers together to synthesize hydrophilic porous polymers through HIPE. As opposed to HEMA, the fluorinated polyHIPEs made of hexa fluorobutyl acrylate (HFBA) has proved to be high performance and hydrophobic (Azhar et al., 2017). Fluorine atoms in the fluoropolymers impart physicochemical properties superior to conventional polymers in various prospects, for example, oxidative/chemical stability, compatibility with solvents, optical transparency, and environmental stability (Huang et al., 2005). The synergistic effect between the surface chemistry and surface architecture allow the fluorinated polyHIPEs to possess superhydrophobicity (water contact angle > 150°) (Wu et al., 2018).
Water absorption is proved to be poor for hydrophobic polyHIPEs, hence the combination of both hydrophobic and hydrophilic monomers to make highly porous and water absorptive polyHIPEs has been researched in many reports (Li et al., 2008; Gitli and Silverstein, 2011). Researchers have devised different routes to prepare these types of polyHIPEs. In one route, hydrophobic polyHIPEs are first prepared and then modified to increase hydrophilicity by using second synthesis stage (Livshin and Silverstein, 2009). In another route, water absorbing polyHIPEs are directly synthesized with in W/O HIPEs using single synthesis stage. In this route hydrophilic and hydrophobic monomers are simultaneously polymerized the droplet phase and continuous phase, respectively (Ruckenstein and Park, 1990). All these reports described only hydrophilic nature of resulting polyHIPEs, and no one discussed hydrophobic characteristics and morphological details of these polyHIPEs.
As our research group’s main focus is on fluorinated materials including novel fluoropolymers and surfactants (Wan et al., 2018; Xu et al., 2017; Zhang et al., 2018), here in, we present a solution to stabilize MMA based HIPEs via fluorinated di-block copolymer (FDB) even with addition of hydrophilic and hydrophobic co-monomers. FDB gave superb stabilization to these HIPEs so they bear high temperature requirement of free radical polymerization without phase separation. Even after inclusion of highly hydrophilic HEMA and hydrophobic HFBA as co-monomer in the main formulation, the stabilization was not affected. Addition of HFBA enabled these polyHIPEs to be used in oil/water separation by virtue of high interconnectivity, hydrophobicity and oleophilicity. It is to be noted that addition of co-monomers in current research was not to help in stabilization of MMA HIPEs because the stabilization of these emulsions were achieved by addition of solely di-block copolymer (FDB). Effect of monomers types and their concentration on wettability, thermal stability and morphology of MMA based polyHIPEs were studied in detail.
2 Experimental section
2.1 Materials
2-hydroxyethyl methacrylate (HEMA, 97%) was purchased from Aldrich. 2-(Dimethylamino)ethyl methacrylate (DMAEMA, 99%) was supplied by Shanghai Macklin Biochemical Co. Ltd. and flushed through alkaline alumina column to remove inhibitor. Trifluoroethyl methacrylate (TFEMA), divinyl benzene (DVB, 80%), and methyl methacrylate (MMA) were purchased from Aladdin. Both TFEMA and MMA were distilled under reduced pressure and stored in refrigerator prior to use. Hexafluorobutyl acrylate (HFBA) was provided by Fluorine Silicon Chemical Company and also passed through basic Al2O3 column to get rid of inhibitor. Tetrahydrofuran (THF) and 1,4-Dioxane were bought from Sinopharm Chemical Reagent Co. Ltd. Calcium carbonate di-hydrated (CaCl2·2H2O) was supplied by Shanghai Zhanyun Chemical Co. Ltd. 2,2′-azobisisobutyronitrile (AIBN) was purchased from Lingfeng Chemical Reagent Co. Ltd. n-Hexane (>97%) was provided by Tianjin Fuyu Chemical Company. Deionized water (DI) was used in all experiments. Cumyl dithiobenzoate (CDB-RAFT agent) was synthesized by following previous literature report (Yang Liu et al., 2005).
2.2 Synthesis of fluorinated di-block copolymer PDMAEMA-b-PTFEMA (FDB)
Synthesis of FDB was performed in two steps. Firstly, macro-chain transfer agent PDMAEMA was synthesized by following our previous literature report using RAFT polymerization (Azhar et al., 2017). Typically, DMAEMA (7.4 g; 47.07 mmol), CDB (0.1282 g; 0.47 mmol), AIBN (0.0286 g; 0.17 mmol) with CDB/AIBN molar ratio; 2.7, and 1,4-dioxane (12.3 g) were mixed in a 50 ml round bottom flask with the help of magnetic stirrer. Then the reaction flask was sealed and purged with nitrogen gas and placed in a preheated water bath with continuous magnetic stirring at 70 °C for 14 h. Then the reaction was quenched in ice water. The resulting homopolymer was extracted and purified by precipitation into excess cold n-hexane and the sticky product was vacuum dried at 30 °C for 24 h. In the second synthesis step, as prepared first block was utilized to prepare fluorinated di-block copolymer (FDB). Typically, PDMAEMA (5.2220 g; 0.3926 mmol), AIBN (0.0215 g, 0.13 mmol) with PDMAEMA/initiator molar ratio = 3 and TFEMA (6.6 g; 39.26 mmol) were dissolved in (Dioxane; 13.16 g) using 50 ml round bottom flask. The reaction mixture was sealed and purged with nitrogen gas to completely eliminate oxygen from vessel and placed on water bath at 65 °C for 16 h with continuous magnetic stirring. Then the reaction vessel was placed in ice water in order to quench the reaction followed by precipitation of resulting copolymer into excess cold n-hexane. After that the product was filtered from organic solvent and vacuum dried at 35 °C for 24 h.
2.3 MMA-HEMA hydrophilic polyHIPEs preparation
Typical hydrophilic (sample 2; Table 1) polyHIPE was prepared as follows: FDB (0.1960 g) was dissolved in MMA (2.376 g) and HEMA (0.594 g) through ultra-sonication for 10 min using 20 ml glass vial. Then, DVB (0.3564 g) as cross linker and AIBN (0.0356 g) as an initiator was added in to the mixture. The reaction vessel was again placed in ultra-sonication apparatus for 5 min to ensure complete mixing of organic phase. Organic phase was poured into 3 necked round bottom flask equipped with overhead mechanical stirrer. Then the aqueous phase 14.4 g (0.2 M CaCl2.2H20 to suppress Ostwald ripening) (Wong et al., 2013) was drop wise added into the organic phase under constant mechanical stirring at 450 RPM. After complete addition of the water phase, the HIPE was further agitated for 15 min at 2000 RPM to ensure homogeneity. The part of MMA-HEMA HIPE was immediately taken on a glass slide for optical microscopy and remaining was taken in centrifugal tube (as a monolithic mold), and then placed in a convection oven for free radical polymerization at 60 °C for 18 h. At last, MMA-HEMA polyHIPEs were taken out from molds and washed through soxhelet extraction using isopropanol followed by air drying in an oven at 55 °C until constant weight. Sample 1 and sample 8 were prepared without addition of co-monomer. Samples 2–4 and sample 9 was prepared with addition of hydrophilic co-monomer of HEMA, using DVB and EGDMA as cross-linkers respectively.
ID
MMA (wt %)a
HEMA (wt %)b
HFBA (wt %)b
FDB (wt %)c
DVB (wt %)c
EGDMA (wt %)c
Specific surface area (m2/g)d
Porosity (%)e
HIPE stability (h)
Morphologyf
1
100
0
0
5.5
10
0
18.98 ± 2.2
81.3
>15
Regular
2
80
20
0
5.5
10
0
17.80 ± 1.6
80.9
>36
Regular
3
60
40
0
5.5
10
0
17.11 ± 2.3
79.7
>36
Regular
4
40
60
0
5.5
10
0
–
–
ISj
–
5
80
0
20
5.5
10
0
19.3 ± 1.1
80.3
>36
Regular
6
60
0
40
5.5
10
0
18.8 ± 2.4
81
>36
Regular
7
40
0
60
5.5
10
0
19.1 ± 2.1
81.5
>36
Regular
8
100
0
0
5.5
0
10
18.21 ± 3
79.6
<12
Regular
9
80
20
0
5.5
0
10
17.1 ± 2.5
82.4
<12
Irregular
10
80
0
20
5.5
0
10
17.9 ± 3.4
81.2
<12
Regular
2.4 MMA-HFBA hydrophobic polyHIPEs preparation
The synthesis steps for hydrophobic MMA-HFBA polyHIPE were same as that of hydrophilic polyHIPE, just by replacing co-monomer of HEMA with HFBA. Typical sample 5 was prepared in 20 ml glass vial. FDB (0.1960 g), MMA (2.736 g) and HFBA (0.594 g) were dissolved using ultra-sonication for 10 min. Then, DVB (0.3564 g) as cross linker and AIBN (0.0356 g) as an initiator was included in the mixture. The vial was again shifted in ultra-sonication bath for 5 min to ensure complete mixing of organic phase components. Then the prepared mixture was poured into 3 necked round bottom flask equipped with overhead mechanical stirrer. Then the aqueous phase 80.2 wt% containing (0.2 M CaCl2.2H20) was drop wise added into the external phase under constant mechanical agitation at 450 RPM. When all the internal phase was added, the MMA-HFBA HIPE was further stirred for 15 min at 2000 RPM to ensure homogeneity. The part of HIPE was instantly taken on a glass slide for optical microscopy and remaining was poured in centrifugal tube (as a monolithic mold), and then placed in an oven for polymerization at 60 °C for 16 h. Finally, MMA-HFBA polyHIPEs were taken out from molds and washed through soxhelet extraction using isopropanol followed by convective drying in an oven at 55 °C until constant weight. Samples 5–7 and sample 10 were prepared with addition of hydrophobic co-monomer of HFBA using DVB and EGDMA as cross-linkers.
3 Characterization
3.1 1H NMR Spectroscopy
Bruker Advance III 400 MHz nuclear magnetic resonance spectrometer was used to record 1H NMR spectra at room temperature using DMSO and CDCl3 for PDMAEMA and FDB, respectively. Samples were prepared separately in two NMR tubes by dissolving a trace amount of macro-CTA and di-block copolymer in the solvents.
3.2 Gel permeation chromatography (GPC)
The dispersity (Ð) and number average molecular weight (Mn) of PDMAMEA and FDB was analyzed by gel permeation chromatography (GPC) (Waters 1500) equipped with HPLC pumps and refractive index detector. Monodisperse polystyrene standards with molecular weight range 1.18 × 103 to 2.25 × 106 g mol−1 by Shodex were used for molecular weight calibration. THF was used as an eluent at a flow rate of 1 ml/min. Samples were injected into the GPC apparatus at a concentration of 3 ml/L after passing through porous membrane of size 0.45 μm. Theoretical molecular weights were calculated with respect to 70% conversion.
3.3 Optical Microscopy
Optical microscopy was performed on Nikon Eclipse LV100POL, Japan. The droplets of HIPE were spread on glass slide immediately after its preparation prior to optical microscopy. At least 150 droplets were taken for measurements of droplet size distribution of every HIPE sample in Nano Measurer 1.2 software.
3.4 Scanning electron microscopy (SEM)
The inside morphology of the both hydrophobic and hydrophilic MMA polyHIPEs were investigated by scanning electron microscope (SEM) (S-2500, Hitachi Seiki ltd., Japan). Carbon sticker was used to fix the polyHIPE sample (each of 0.5 cm3 in size) on aluminum stub. After that, samples were sputtered with gold (Scan Coat Six SEM Sputter Coater, Edwards, Ltd., Crawley, United Kingdom) at 20 mA for 70 s in order to ensure good electrical conductivity. We also considered the possible variation in porous morphology due to the HIPE droplet coalescence and sedimentation, so that images were taken from top, middle and bottom sections of the samples. Nano Measurer 1.2 software was used to estimate pore and pore throat dimensions from SEM images and at least 150 pores were taken in measurements of every polyHIPE image. A statistical correction factor was applied (Toledo and Urbano, 2016) to all measurements due to the underestimation of real values, the correction factor is given in Eq. (1):
3.5 Specific surface area (SSA), porosity and openness
Specific surface areas of polyHIPEs were analyzed by nitrogen adsorption isotherm using Brunaur-Emmett-Teller (BET) model with Micromeritics TriStar II 3020. Approximately 200 mg of each sample was heated at 160 °C for 24 h to remove contaminants prior to adsorption of the gas. Porosities of the samples were calculated by the liquid displacement test (Xu et al., 2014).
3.6 Water uptake (WU) measurements
Water uptake experiments were performed as a function of time for the prepared samples. Dried poly(MMA-HEMA) were weighed (w1) and placed in a closed vial containing DI water. The samples after water absorption were weighed periodically (w2). Excess water on the surface of polyHIPE was wiped off with a filter paper. The water uptake was calculated according to the given Eq. (2):
3.7 Contact angle measurements
Hydrophobicity of the MMA based polyHIPEs were measured by a contact angle instrument OCA drop shape analyzer (Data Physics Co., Germany) at room temperature. Contact angles were measured at five different places on each sample and then the average value was taken as final.
3.8 Oil adsorption test
0.25 g (approximately 20 mm in diameter and 10 mm in height) of polyHIPE foam in cylindrical shape was placed into the oil/water mixture where oil floated on the surface of water. Oil intake capacity k was calculated by the following Eq. (3):
4 Results and discussions
4.1 Characterization of fluorinated di-block copolymer (FDB)
Two steps RAFT solution polymerization was performed to synthesize fluorinated di-block copolymer (PDMAEMA-b-PTFEMA). Firstly, the structure of FDB was confirmed by 1H NMR spectroscopy as demonstrated in Fig. 1. For the hydrophilic PDMAEMA block, the characteristic resonance peak at δ = 2.34 ((CH3)2NCH2CH2—) (c H), δ = 2.55 ((CH3)2NCH2CH2—) (d H) and δ = 4.04 ((CH3)2NCH2CH2—) (e H) were observed (Chen et al., 2015). For the hydrophobic fluorinated block of PTFEMA, the feature peak at δ = 4.39 were assigned to CH2 in (—OCH2CF3)(g H) (Liu et al., 2008). All the characteristic peaks assigned to PDMAEMA and FDB protons were evidently displayed in nuclear magnetic resonance spectra.
1H NMR of PDMAEMA (bottom) and PDMAEMA-b-PTFEMA (top).
GPC was employed to characterize molecular weight and its distributions of PDMAEMA and PDMAEMA-b-PTFEMA. The number average molar mass (Mn) of first block came out to be 11,415 g/mol with dispersity (Ð) of 1.17, and Mn of FDB was 13,301 g/mol with Ð of 1.30. The representative chromatograms of resulting polymers as a function of elution time are depicted in Fig. 2. Only a single peak appeared for both first and di-block copolymer. It indicated that the product was composed of amphiphilic block copolymers of PDMEAMA-b-PTFEMA rather than a mixture containing homopolymers of PDMAEMA and PTFEMA (Chen et al., 2015). Peak shifting towards higher molecular weights also proved to be successful in the synthesis of FDB. The theoretical molecular weights of PDMAEMA and PDMAEMA-b-PTFEMA were calculated to be 11,196 g/mol and 22,963 g/mol, respectively.GPC curves of PDMAEMA and PDMAEMA-b-PTFEMA.
Furthermore, FT-IR (Chen et al., 2015; Li et al., 2014) analysis was executed to characterize the detailed structure of resulting first and di-block copolymers (Fig. 3). The peaks at 2951 cm−1 and 2860 cm−1 were designated to characteristic stretching vibrations of methyl (—CH3) and methylene (—CH2—) groups, respectively. The peak at 2770 cm−1 was assigned to the characteristic stretching of carbon-hydrogen (C—H) bonds in —N(CH3)2. C⚌O stretching vibration peak was noticed at 1726 cm−1. The bending vibration of CH2 groups appeared at 1453 cm−1. The peak at 1147 cm−1 was attributed to the stretching vibration absorption peak of C—O. The band at 655 cm−1 was assigned to the band vibration of C—F in CF3 (Li et al., 2014). Moreover, none of the peaks were observed in between the range of 1680–1640 cm−1 which is the range of C⚌C bond, suggested that monomer was fully consumed during RAFT polymerization process (Papadopoulou et al., 2011). Spectrum of PDMAEMA macro RAFT agent is shown above to the spectrum of FDB in Fig. 3. It can be clearly observed that none of the peak appeared in the region between 640 cm−1 and 680 cm−1 (Fig. 3: PDMAEMA spectrum). Therefore, FT-IR results also proved that both hydrophilic (DMAEMA) and hydrophobic block (TFEMA) had effectively taken part in polymerization process and PDMAEMA-b-PTFEMA copolymer was successfully synthesized.FT-IR of PDMAEMA and PDMAEMA-b-PTFEMA.
4.2 HIPE stability and optical microscopy
Stability of HIPEs is of vital importance because if the emulsions are stable enough to bear the elevated temperatures at least until gelation point, only then they can form the resultant polyHIPE, which are useful for practical applications (Barbetta and Cameron, 2004; Williams, 1991). For the stability of HIPEs, a suitable surfactant is a key factor. It is worth-noticeable that not every kind of surfactant is suitable for HIPE stabilization (Wan et al., 2018). Especially, monomers with intermediate hydrophilicity are very difficult to form HIPE and follows phase separation immediately even during the preparation process, due to their water soluble nature. Here, HIPEs and their representative polyHIPEs of MMA with HEMA (Scheme 1) and HFBA (Scheme 2) as co-monomers are prepared by using FDB as a sole stabilizer.Reaction scheme of preparation of Poly(MMA-HEMA).
Reaction scheme of preparation of Poly(MMA-HFBA).
The emulsions were proved to be highly stable against coalescence even when exposed to the elevated temperatures for free radical polymerization and gave rise to polymeric monolithic polyHIPEs as shown in Fig. 4a and b. The reason of this obtrusive stability provided by FDB can be attributed to the strong hydrophobic segment in its structure, which provides a powerful anchoring layer of di-block copolymer at monomer/water interface. This layer actually prevents hydrophilic as well as hydrophobic monomers and co-monomers to mix in the water phase. Thus, the di-block copolymer containing fluorine is proved to be suitable for stabilization hydrophobic as well hydrophilic emulsion systems.Photographs of (a) MMA-HEMA HIPE and its polyHIPE, (b) MMA-HFBA HIPE and its polyHIPE.
In order to investigate the droplet sizes and their distribution, the optical microscopy was performed for the prepared emulsions (sample 1–3, 5 and 6 Table. 1). Sample 4 was phase separated immediately after HIPE preparation, so it was not taken for optical microscopy. As shown in Fig. 5, discrete droplets with clear interfacial boundaries are present in all HIPEs, which demonstrate the superb stabilization ability of FDB by making distinct droplets in emulsion formation. Next, effect of varying co-monomers concentration was investigated. When amount of hydrophilic co-monomer HEMA was 20 wt% in MMA based HIPEs, the droplet sizes were smaller and the droplet size distribution was pretty narrower between the ranges of 2–8 µm. When the amount of HEMA was increased to 40 wt% the sizes of the HIPE droplets turned bigger and the droplet size distribution was in between the range of 5–18 µm.Optical microscopy images and droplet size distribution plots (a) sample 1, (b) sample 2, (c) sample 3, (d) sample 5, and (e) sample 6, scale bar 25 µm.
The reason of increase in sizes of the droplets upon increasing the hydrophilic co-monomer can be attributed to the increase in miscibility of monomers and water phase that resulted in Ostwald ripening. When HEMA concentration was increased then miscibility of oil phase droplets in water took place which finally merged to form comparatively bigger droplets (Zhang et al., 2009).
When the hydrophobic monomer HFBA was incorporated in emulsion formulation, then both the sizes and distribution of the droplets were on higher side as compared to their hydrophilic counter-parts. When the amount of HFBA was 20 wt%, the droplet size distribution was observed to be in the range of 3–19 µm and most of the droplets were in the size range of 12–17 µm. Overall sizes of the droplets were decreased upon further addition of hydrophobic monomer at 40 wt%. Almost same size distribution range was found as observed in 20 wt%, but most of the droplets were in the range of 3–9 µm. The reason of decrease in size of the droplets may be due to the good stability of the emulsion provided by fluorinated di-block copolymer to the fluoromonomer (HFBA). As it is reported in literature that fluoro-compatibility between monomer and surfactant can be helpful in stabilization of fluorinated-HIPEs (Azhar et al., 2017). Thus, when the concentration of HFBA was increased as compared to the partially hydrophilic monomer MMA, the FDB stabilization effect was ultimately increased as fluorine atoms were present in the monomer, which caused to form discrete droplets.
4.3 Morphology of MMA based hydrophilic and hydrophobic polyHIPEs
Size, porosity and interconnectivity of polyHIPEs are important parameters to be considered for their use in practical applications such as separation membranes, reaction supports for catalysts, column for chromatography and many biological applications (Silverstein, 2014). In the present research, HIPEs stabilized by FDB with the assistant of free radical initiator were further allowed to polymerize at 60 °C. All HIPEs were stable enough to withstand at high temperatures and finally gave rise to solid monoliths. The obtained monoliths were then taken out from the molds and air-dried. The morphology of the polyHIPEs was characterized by SEM as shown in Fig. 6. It is interesting to note that polyHIPEs morphologies were similar to that of respective HIPEs (in Fig. 5), and no distinguished changes in size of the pores were observed in both. This means that HIPEs containing FDB as stabilizer, MMA as monomer, HEMA and HFBA as co-monomers system is very effective against coalescence due to suppression of Ostwald ripening phenomena (which could had been the possible cause of change in sizes of the droplets). Hydrophilic poly(MMA-HEMA) demonstrated BET specific surface areas in the range of 17.11 ± 2.3 to 17.8 ± 1.6 m2/g with porosities values from 79.7 to 80.9%. On the other hand, when hydrophobic co-monomer was added then a slight increase in specific surface area (SSA) values and porosities were observed. Porosities values increased from 80.3 to 82.5% and Brunaur-Emmett-Teller (BET) SSA values from 18.8 ± 2.4 to 19.3 ± 1.1 m2/g.SEM images of (a) sample 1, (b) sample 2, (c) sample 3, (d) sample 5, and (e) sample 6, big scale bar 50 µm and inset scale bar 5 µm.
Effects of hydrophilic and hydrophobic co-monomers on morphology of resulting polyHIPE materials are described here. As shown in Fig. 6a, without the addition of any co-monomer, the MMA HIPE showed highly interconnected and porous morphology. When 20 wt% hydrophilic co-monomer HEMA was added into the HIPE, then the resulting polyHIPE was found to decrease in pore size with almost the same level of porosity as that of MMA HIPE, as shown in Table 1 and Fig. 5b. When the amount of HEMA was increased from 20 wt% to 40 wt%, the increases in size of the pores were detected (Fig. 6c). Further increase in HEMA weight percent did not give any emulsion (Table 1, sample 4). This may be due to the stabilization ability of FDB which is good for MMA monomer (partially hydrophobic), but not as effective for the system with fully hydrophilic monomers such as HEMA. So, when the amount of HEMA was increased up to the certain critical level, the ability of the di-block to emulsify the oil phase at interphase of oil and water was minified, which led the small droplets of the emulsion to merge with each other to form bigger size droplets. On the other hand, when the hydrophobic co-monomer HFBA was incorporated into the HIPE, then the resulting polyHIPE morphology demonstrated bigger size pores along with irregular shape of pore throats. These bigger pore sizes and interconnections are very attractive materials for oil-water separation applications (Zhang et al., 2016).
EGDMA is the commonly used cross-linker for MMA based materials in literature (Huš and Krajnc, 2014), so we also checked the compatibility of FDB to prepare polyHIPEs of MMA, HEMA and HFBA via HIPE cross-linked with EGDMA. It was noted that all types of HIPEs did not get phase separation when exposed to elevated temperatures and the final polyHIPE materials were obtained as per desired monolithic shape. The morphology of these polyHIPEs is given in Fig. 7. It is clear from the figure that regular polyHIPE morphology was obtained in the case of MMA and MMA-HFBA. But when HEMA was employed as co-monomer, the loss of regular polyHIPE morphology was evident. These results suggest that EDGMA as a cross-linker is not a suitable choice for obtaining regular polyHIPE morphology when using FDB as sole stabilizer. This loss of polyHIPE morphology can be attributed to the very high miscibility of hydrophilic cross linker EGDMA with HEMA which may minify the effect of di-block copolymer at oil-water interfacial layer to form the regular shape of droplets.SEM images of polyHIPEs prepared with EGDMA as cross-linker (a) sample 8, (b) sample 9, and (c) sample 10, big scale bar 100 µm and inset scale bar 10 µm.
Average pore sizes of the hydrophilic poly(MMA-HEMA) till 20 wt% HEMA was almost same as that of the pure MMA HIPE i.e. 7 ± 1.4 µm. After 20 wt%, an increase in the average pore size of the polyHIPE was observed. The values of the average pore sizes of 11 ± 2.7 µm were found at 40 wt% HEMA co-monomer. After that concentration, monomer-water system was not stable enough to form HIPE. Addition of hydrophobic monomer demonstrated bigger average pore sizes as compared to both poly(MMA) and poly(MMA-HEMA). Average sizes were calculated in the range of 12 ± 1.6 µm and 12.7 ± 1.2 µm for concentration of HFBA 20 wt% and 40 wt%, respectively.
4.4 Thermal properties and wettability of polyHIPEs
Thermogravimetric analysis (TGA) was performed to investigate the thermal stabilities of poly(MMA) (sample 1), poly(MMA-HEMA) (sample 3) and poly(MMA-HFBA) (sample 5). Respective thermograms are presented in Fig. 8. Poly(MMA) exhibited a degradation onset temperature of 347 °C at the expense of 10% weight loss. When hydrophilic monomer was introduced into the HIPE, then, resulting poly(MMA-HEMA) followed a decreasing trend and showed onset degradation temperature of 305 °C. Surprisingly, addition of hydrophobic co-monomer in MMA-HIPEs increased onset degradation temperature from 305 to 360 °C, as compared to their hydrophilic counterparts. The possible reason of increase in thermal stability is the presence of fluorine atoms in the polymer chains. As it is well known in literature that fluorine atoms impart some very special properties such as thermal resistance, optical transparency, chemical and oxidative stabilities to the polymers in which they are used (Huang et al., 2005).TGA of MMA based polyHIPEs.
Hydrophobic porous polymeric materials are suitable choice for water-oil separation, whereas hydrophilic polymers are extensively used in biomedical fields such as tissue engineering. It is well known that increase in hydrophilicity results in increasing the biocompatibility of the polymer blend surface. Therefore, increasing surface hydrophilicity may positively affect the interaction between tissues and the surface which ultimately decreases the friction between them (Rezaei and Mohd Ishak, 2011). In view of the above mentioned very important practical applications, hydrophobic/hydrophilic properties of the prepared polyHIPEs are analyzed in this report. When the drops of deionized water were dripped on the surface of poly(MMA-HEMA) (Table 1, sample 3), these were absorbed by the poly(HIPE). The demonstration of the droplet absorption is shown in Fig. 9a and video is being provided as supplementary information (S1-video). When the same droplet test was performed on poly(MMA-HFBA), the water droplet did not penetrate into the polymer network and formed a spherical shape on the surface as illustrated in Fig. 9b and S2-video. Absorption of droplets in the poly(MMA-HEMA) is attributed to the superb hydrophilic potential of pendant hydroxyl groups present in HEMA (Okano et al., 1978). On the other hand, the droplet stay on the surface of polyHIPE with HFBA as co-monomer is due to the fluorine atoms present in the structure. As it has been proved that fluorine atoms are responsible for preparation of many hydrophobic (>90° WCA < 150°) and super hydrophobic materials (WCA > 150°), where WCA is water contact angle (Ma and Hill, 2006).
Video 1
Video 1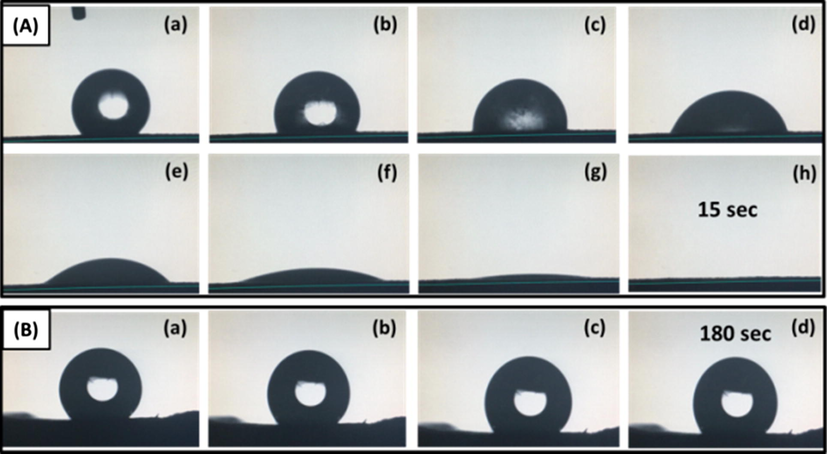
- Wettability of hydrophilic and hydrophobic polyHIPEs: (A) water droplet on hydrophilic poly(MMA-HEMA), and (B) water droplet on hydrophobic poly(MMA-HFBA).
Video 2
Video 2In order to further verify localization of atoms on the surface of the hydrophobic polyHIPEs, EDS analysis was performed. The results are shown in Fig. 10. It can be clearly seen that fluorine atoms are present on the surface of the sample, which imparts special properties to resulting polyHIPEs such as thermal stability and hydrophobicity.EDS analysis of poly(MMA-HFBA) (confirmation of fluorine atoms localization).
4.5 Oil adsorption of hydrophobic poly(MMA-HFBA)
PolyHIPEs are promising materials for oil-water separation owing to their high porosity and specific surface areas. One of the utmost important requirements for oil-water separation is the hydrophobicity of the materials used for adsorption of oil. If the polyHIPE is hydrophobic then it can allow oil to adsorb in the pores and at the same time prohibit water to enter in these materials.Owing to the inclusion of fluorinated co-monomer in partially hydrophilic monomer MMA, the resulting polyHIPEs turned hydrophobic. Hence these hydrophobic polyHIPEs were utilized to adsorb oil from the surface of the water.
Oil adsorption demonstration is provided in Fig. 11. When poly(MMA-HFBA) were added into the oil-water mixture, the oil was rapidly adsorbed by the polyHIPEs, as shown in Fig. 11a–c and S3-video. It can be clearly observed in Fig. 11d, that when oil was dripped on the poly(MMA-HFBA) it penetrated into the pores and when water was dripped it turned into spherical droplets on the surface, revealing its simultaneous hydrophobic and oleophilic nature. Water contact angle of these spherical droplets were measured to be 139° (Fig. 11e). Oil adsorption capacities towards various organic oils are depicted in Fig. 11f. Interestingly, the poly(MMA-HFBA) adsorbed almost 740% of the oil (for dichloromethane) as compared to the initial weight. These significant oil adsorption capacities can be attributed to the high porosity and specific surface areas of these interconnected polyHIPEs, as shown in Table 1 (Azhar et al., 2017). Poly(HFBA-DVB) foams have been proven as high performance materials, which can be used in extreme conditions, due to the presence of fluorine in the polymeric network (Azhar et al., 2017). It is to be noted that in the present research, fluorine is also incorporated as co-monomer in the poly(MMA-HFBA), hence it could impart high performance characteristic as well. These materials would find promising applications in environmental engineering.
Video 3
Video 3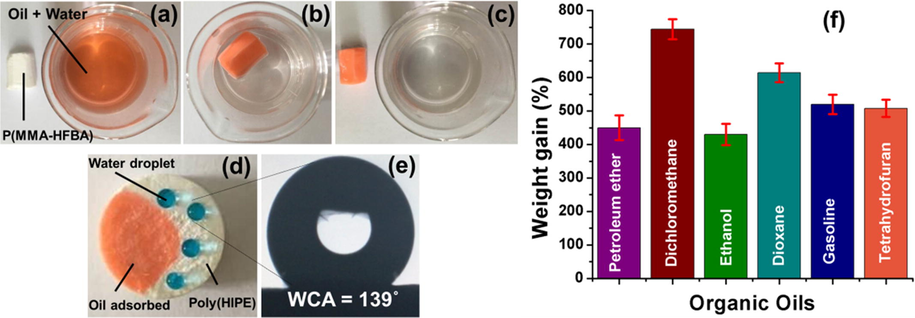
- (a–c) Oil-water separation demonstration, (d) simultaneous hydrophobicity and oleophilicity of poly(MMA-HFBA), (e) Water contact angle, and (f) Adsorption capacity towards various organic oils.
4.6 Water uptake properties of poly(MMA-HEMA)
PolyHIPEs are very attractive materials for water as well as hazardous contaminants adsorption (Kovačič et al., 2018). A quick absorption can be achieved with-in the pores by displacement of the air through a simple dousing of these materials into targeted liquids (Sergienko et al., 2002). Water absorption can take place in two steps with-in the porous polymeric materials. Initially, water diffuses into the pores owing to the wettability of the porous walls with in the first hours of contact with water. Then, in the second step, diffusion of the water molecules into the polymeric network took place with slow speed due to the relaxation of polymeric chains (Toledo and Urbano, 2016). The HEMA based hydrogels have been used for the water absorption but their practical use is hampered due to very weak mechanical strength. On the other-hand, polyMMA HIPEs have been approved by FDA to be used in water absorption application due to enhanced mechanical properties as compared to fully hydrophilic counterparts such as HEMA (Serrano-Aroca and Llorens-Gámez, 2017). Pre-polymerization functionalization method for the preparation of GMA-based hydrogel polyHIPEs was proposed to increase the water uptake capacity of polyHIPEs (Pahovnik et al., 2016). Double network (DN) hydrogels were prepared to improve water retaining and compressive strength (Kovačič and Silverstein, 2017). We have successfully obtained HIPE by combining both partially hydrophobic MMA and fully hydrophilic monomer HEMA with the help of FDB as stabilizer. These HIPEs were able to withstand elevated temperatures and porous polyHIPEs were formed as result. This section is aimed to demonstrate significant application of MMA based HIPEs when using HEMA as co-monomer. Contrary to the HFBA, when HEMA was used as co-monomer the resulting polyHIPEs were hydrophilic in nature. Initially, water was allowed to absorb in the MMA-HEMA polyHIPEs with DVB as cross-linker (sample 5), but the absorption capacity and absorption rate was not high. This might be due to the hydrophobic cross-linker DVB in to the polymeric network. When hydrophilic cross-linker EGDMA based MMA-HEMA polyHIPEs were employed to absorb water, the absorption capacity as well as absorption rate were significantly improved. It is to be noted that although porous material with semi open morphology was achieved in DVB based polyHIPEs but still the water absorption was higher in the EGDMA based MMA-HEMA polyHIPEs instead of irregular polyHIPE morphology. Hence, in this case the hydrophilic and hydrophobic nature of cross-linker effected more than that of regular porous morphology of polyHIPE.
Water absorption performances of both co-monomers HFBA and HEMA based MMA polyHIPEs were tested. As shown in Fig. 12, when HFBA was used as co-monomer the water absorption was approximately equal to none with straight line at 0 g/g uptake. On contrary, when HEMA as co-monomer was used in the same MMA HIPEs the resultant porous polymer demonstrated water uptake up to around 2.50 g/g of polyHIPE. It was due to pendant –OH groups present in the main structure which gave water absorption capability to HEMA.Water uptake capacity of poly(MMA-HEMA) and poly(MMA-HFBA).
5 Conclusions
In this study, we reported a fluorinated di-block copolymer which enabled the stabilization of very difficult to emulsify MMA monomer in water. HEMA and HFBA were employed as co-monomers in the emulsion in order to impart various properties such as thermal stability, wettability and morphology of the resultant polyHIPEs. FDB molecules anchored well at the oil-water interface of water-in-oil emulsion improved the hydrophobicity of HIPEs, which in turn prevented the droplets from mixing with monomers. Stability of FDB stabilized MMA based HIPEs were up to more than 36 h. By increasing the concentration of more hydrophilic co-monomer in the emulsion, the pore sizes were observed to be increased from 7 ± 1.4 to 11 ± 2.7 µm. Addition of HFBA comparatively increased the over all sizes of the pores and imparted hydrophobicity in the final polyHIPE (WCA = 139°). Also thermal stabilities of poly(MMA-HFBA) were significantly improved from 305 to 360 °C as compared to poly(MMA-HEMA). Finally, polyHIPE monoliths were tested to demonstrate their useful applications in oil-water separation and water uptake, respectively. Hydrophobic polyHIPEs adsorbed the organic oil upto 740%, while hydrophilic polyHIPEs absorbed water to approximately 2.50 g/g of the weight of monolith. Over all, the idea of using fluorinated di-block copolymers in stabilization of hydrophobic as well as hydrophilic monomers was successful and resultant polyHIPEs would have significant applications in polymer engineering, environmental and biological sciences.
Author contributions
Umair Azhar and Rimsha Yaqub contributed equally to this work. The manuscript was written through contributions of all authors. All authors have given approval to the final version of the manuscript.
Acknowledgement
The work was financed by the Scientific Research Project of Shandong Provisional Department of Education [Grant No. J11LB02], Natural Science Foundation of Shandong Province [Grant No. ZR2017ZC0529], Young Taishan Scholars tsqn [Grant No. 20161036], the National Science Foundation of China [Grant No. 21304037], and National Science Foundation of China [Grant No. 21675064].
Data availability
Readers can obtain the research data required to reproduce the work reported in the manuscript from the journals home page in the form of original article.
References
- A cationic fluorosurfactant for fabrication of high-performance fluoropolymer foams with controllable morphology. Mater. Des.. 2017;124:194-202.
- [Google Scholar]
- Porous multifunctional fluoropolymer composite foams prepared via humic acid modified Fe3O4 Nanoparticles stabilized Pickering high internal phase emulsion using cationic fluorosurfactant as co-stabilizer. Arabian J. Chem.. 2019;12:559-572.
- [Google Scholar]
- Morphology and surface area of emulsion-derived (PolyHIPE) solid foams prepared with oil-phase soluble porogenic solvents: Span 80 as surfactant. Macromolecules. 2004;37(9):3188-3201.
- [Google Scholar]
- High internal phase pickering emulsions stabilized solely by peanut protein microgel particles with multiple potential applications. Angew. Chem. Int. Ed. 2018
- [Google Scholar]
- Emulsion-derived foams (PolyHIPEs) containing poly(E-caprolactone) as matrixes for tissue engineering. Biomacromolecules. 2001;2:154-164.
- [Google Scholar]
- High internal phase emulsion templating as a route to well-defined porous polymers. Polymer. 2005;46(5):1439-1449.
- [Google Scholar]
- Synthesis of a cationic fluorinated polyacrylate emulsifier-free emulsion via ab initio RAFT emulsion polymerization and its hydrophobic properties of coating films. RSC Adv.. 2015;5(118):97231-97238.
- [Google Scholar]
- Click chemistry as a means to functionalize macroporous PolyHIPE. Soft Matter. 2009;5(4):804-811.
- [Google Scholar]
- Active growth factor delivery from poly(D, L-lactide-coglycolide) foams prepared in supercritical CO2. J. Control. Release. 2000;66:177-185.
- [Google Scholar]
- First acidic macro-mesocellular aluminosilicate monolithic foams “SiAl(HIPE)” and their catalytic properties. Chem. Commun.. 2015;51(74):13993-14128.
- [Google Scholar]
- Emulsion templated bicontinuous hydrophobic-hydrophilic polymers: loading and release. Polymer. 2011;52(1):107-115.
- [Google Scholar]
- Synthesis and characterization of novel fluoropolymers containing sulfonyl and perfluorocyclobutyl units. Polymer. 2005;46(18):7590-7597.
- [Google Scholar]
- PolyHIPEs from Methyl methacrylate: hierarchically structured microcellular polymers with exceptional mechanical properties. Polymer. 2014;55(17):4420-4424.
- [Google Scholar]
- Hydrogels in healthcare: from static to dynamic material microenvironments. Acta Mater.. 2013;61(3):931-944.
- [Google Scholar]
- Highly porous open-cellular monoliths from 2-hydroxyethyl methacrylate based high internal phase emulsions (HIPEs): preparation and void size tuning. Macromolecules. 2007;40(22):8056-8060.
- [Google Scholar]
- Ring Opening Metathesis Polymerisation (ROMP) as a tool for PolyHIPEs with extraordinary mechanical properties. Acta Chimica Slovenica. 2013;60(2):448-454.
- [Google Scholar]
- Covalent incorporation of the surfactant into high internal phase emulsion templated polymeric foams. Chem. Commun.. 2015;51(36):7725-7728.
- [Google Scholar]
- Hydrogels through emulsion templating: sequential polymerization and double networks. Polym. Chem.. 2017;8(40):6319-6328.
- [Google Scholar]
- Highly porous cationic polyelectrolytes via oil-in-water concentrated emulsions: synthesis and adsorption kinetic study. Langmuir. 2018;34(35):10353-10362.
- [Google Scholar]
- Porous poly (2-hydroxyethyl methacrylate) hydrogels synthesized within high internal phase emulsions. Soft Matter. 2007;3(12):1525-1529.
- [Google Scholar]
- The facile synthesis of PMMA polyHIPEs with highly interconnected porous microstructures. J. Mater. Sci.. 2016;51(19):9005-9018.
- [Google Scholar]
- Synthesis and characterization of fluorinated diblock copolymer of 2,2,2-trifluoroethyl methacrylate and methyl methacrylate based on RAFT polymerzation. J. Fluorine Chem.. 2014;165:132-137.
- [Google Scholar]
- Preparation of hydrophilic/hydrophobic porous materials. J. Colloid Interface Sci.. 2008;323(1):120-125.
- [Google Scholar]
- New process for synthesizing fluorinated polymers in supercritical carbon dioxide. Macromolecules. 2008;41:6987-6992.
- [Google Scholar]
- Enhancing hydrophilicity in a hydrophobic porous emulsion-templated polyacrylate. J. Polym. Sci., Part A: Polym. Chem.. 2009;47(18):4840-4845.
- [Google Scholar]
- One-pot interfacial polymerization to prepare PolyHIPEs with functional surface. Colloid. Polym. Sci.. 2015;293(6):1767-1779.
- [Google Scholar]
- Facile fabrication of hierarchical porous resins via high internal phase emulsion and polymeric porogen. Appl. Surf. Sci.. 2014;305:186-193.
- [Google Scholar]
- Functionalization of 2-hydroxyethyl methacrylate-based polyHIPEs: effect of the leaving group. React. Funct. Polym.. 2016;109:99-103.
- [Google Scholar]
- Preparation of macroporous polyHIPE foams via radiation-induced polymerization at room temperature. Colloid Polym. Sci.. 2013;291(7):1649-1656.
- [Google Scholar]
- Influence of the macromolecular surfactant features and reactivity on morphology and surface properties of emulsion-templated porous polymers. Macromolecules. 2015;48(18):6489-6498.
- [Google Scholar]
- Macroporous poly (ionic liquid)/ionic liquid gels via CO2-based emulsion-templating polymerization. Polym. Chem. 2018
- [Google Scholar]
- Inverse high internal phase emulsion polymerization (i-HIPE) of GMMA, HEMA and GDMA for the preparation of superporous hydrogels as a tissue engineering scaffold. J. Mater. Chem. B. 2016;4(3):450-460.
- [Google Scholar]
- The influence of hydrophilic and hydrophobic domains on water wettability of 2-hydroxyethyl methacrylate-styrene copolymers. J. Appl. Polym. Sci.. 1978;22(2):369-377.
- [Google Scholar]
- High porosity, responsive hydrogel copolymers from emulsion templating. Polym. Int.. 2016;65(3):280-289.
- [Google Scholar]
- Synthesis of hydrogel polyHIPEs from functionalized glycidyl methacrylate. Polym. Chem.. 2016;7(32):5132-5138.
- [Google Scholar]
- Hierarchical macro and mesoporous foams synthesized by HIPEs template and interface grafted route for simultaneous removal of λ-cyhalothrin and copper ions. Chem. Eng. J.. 2016;284:1361-1372.
- [Google Scholar]
- Superhydrophobic surfaces from hydrophobic or hydrophilic polymers via nanophase separation or electrospinning/electrospraying. Colloids Surf., A. 2011;387(1–3):71-78.
- [Google Scholar]
- Synthesis of biodegradable macroporous poly (l-lactide)/poly (ε-caprolactone) blend using oil-in-eutectic-mixture high-internal-phase emulsions as template. ACS Appl. Mater. Interfaces. 2016;8(26):16939-16949.
- [Google Scholar]
- Polymeric surfactants: synthesis, properties, and links to applications. Chem. Rev.. 2015;115(16):8504-8563.
- [Google Scholar]
- The biocompatibility and hydrophilicity evaluation of collagen grafted poly(dimethylsiloxane) and poly (2-hydroxyethylmethacrylate) blends. Polym. Test.. 2011;30(1):69-75.
- [Google Scholar]
- The separation of water–ethanol mixtures by pervaporation through hydrophilic—hydrophobic composite membranes. J. Appl. Polym. Sci.. 1990;40(1–2):213-220.
- [Google Scholar]
- Polymerized high internal-phase emulsions: properties and interaction with water. J. Appl. Polym. Sci.. 2002;84(11):2018-2027.
- [Google Scholar]
- Dynamic mechanical analysis and water vapour sorption of highly porous poly(methyl methacrylate) Polymer. 2017;125:58-65.
- [Google Scholar]
- PolyHIPEs: Recent advances in emulsion-templated porous polymers. Prog. Polym. Sci.. 2014;39(1):199-234.
- [Google Scholar]
- Emulsion & foam templating: promising routes to tailor-made porous polymers. Angew. Chem. 2018
- [Google Scholar]
- Inversion of particle-stabilized emulsions to form high-internal-phase emulsions. Angew. Chem. Int. Ed.. 2010;49(12):2163-2166.
- [Google Scholar]
- Poly(2-hydroxyethyl methacrylate)-based porous hydrogel: Influence of surfactant and SiO2 nanoparticles on the morphology, swelling and thermal properties. Eur. Polym. J.. 2016;81:316-326.
- [Google Scholar]
- Supercritical CO2 mediated functionalization of highly porous emulsion-derived foams: ScCO2 absorption and epoxidation. J. CO2 Util.. 2017;21:336-341.
- [Google Scholar]
- Antagonistic effects between magnetite nanoparticles and a hydrophobic surfactant in highly concentrated Pickering emulsions. Langmuir: ACS J. Surfaces Colloids. 2014;30(18):5064-5074.
- [Google Scholar]
- Mechanical properties and drug release behavior of bioactivated PMMA cements. J. Biomater. Appl.. 2012;26(5):581-594.
- [Google Scholar]
- Highly porous and chemical resistive P(TFEMA–DVB) monolith with tunable morphology for rapid oil/water separation. RSC Adv.. 2018;8(15):8355-8364.
- [Google Scholar]
- Control of uniform and interconnected macroporous structure in polyHIPE for enhanced CO2 adsorption/desorption kinetics. Environ. Sci. Technol.. 2016;50(14):7879-7888.
- [Google Scholar]
- Interconnectivity of macroporous hydrogels prepared via graphene oxide-stabilized pickering high internal phase emulsions. Langmuir. 2016;32:982-990.
- [Google Scholar]
- High internal phase water-in-oil emulsions: influence of surfactants and cosurfactants on emulsion stability and foam quality. Langmuir. 1991;7(7):1370-1377.
- [Google Scholar]
- Hierarchical polymerized high internal phase emulsions synthesized from surfactant-stabilized emulsion templates. Langmuir: ACS J. Surfaces Colloids. 2013;29(20):5952-5961.
- [Google Scholar]
- Preparation of emulsion-templated fluorinated polymers and their application in oil/water separation. J. Polym. Sci., Part A: Polym. Chem.. 2018;56(14):1508-1515.
- [Google Scholar]
- Effects of internal-phase contents on porous polymers prepared by a high-internal-phase emulsion method. J. Polym. Res.. 2014;21(9)
- [Google Scholar]
- Synthesis of fluorinated nanoparticles via RAFT dispersion polymerization-induced self-assembly using fluorinated macro-RAFT agents in supercritical carbon dioxide. RSC Adv.. 2017;7(81):51612-51620.
- [Google Scholar]
- Thermal decomposition of cumyl dithiobenzoate. Macromolecules. 2005;38:10332-10335.
- [Google Scholar]
- Tailoring the morphology of emulsion-based (glycidylmethacrylate-divinylbenzene) monoliths. Eur. Polym. J.. 2014;57:127-136.
- [Google Scholar]
- Stability of high internal phase emulsions with sole cationic surfactant and its tailoring morphology of porous polymers based on the emulsions. Polymer. 2009;50(7):1723-1731.
- [Google Scholar]
- PMMA based foams made via surfactant-free high internal phase emulsion templates. Chem. Commun.. 2009;16:2217-2219.
- [Google Scholar]
- Continuous preparation of polyHIPE monoliths from ionomer-stabilized high internal phase emulsions (HIPEs) for efficient recovery of spilled oils. Chem. Eng. J.. 2017;307:812-819.
- [Google Scholar]
- Assembled block copolymer stabilized high internal phase emulsion hydrogels for enhancing oil safety. Ind. Eng. Chem. Res.. 2016;55(16):4499-4505.
- [Google Scholar]
- Emulsion-templated, macroporous hydrogels for enhancing water efficiency in fighting fires. J. Mater. Chem. A. 2017;5(21):10161-10164.
- [Google Scholar]
- Superhydrophobic P (St-DVB) foam prepared by the high internal phase emulsion technique for oil spill recovery. Chem. Eng. J.. 2016;298:117-124.
- [Google Scholar]
- Synthesis of well-defined PVDF-based amphiphilic block copolymer via ITP for antifouling membrane application. Ind. Eng. Chem. Res. 2018
- [Google Scholar]
- Hydrophilic porous polymers based on high internal phase emulsions solely stabilized by poly(urethane urea) nanoparticles. Polymer. 2010;51(16):3612-3617.
- [Google Scholar]