Translate this page into:
Hollow sphere TiO2: Synthesis and its application for dye-sensitized solar cells
* Corresponding author: E-mail address: indriana@ugm.ac.id (I. Kartini)
-
Received: ,
Accepted: ,
Abstract
The architecture of semiconductor oxides as the core photoanodes in the dye-sensitized solar cells affecting the energy conversion of the dye-sensitized solar cells (DSSC) has been a slightly neglected parameter. Herein, we study that the morphology of hollow sphere (HS) TiO2 as the semiconductor photoanode of DSSC has proven to result in the power conversion efficiency (PCE) higher than the solid sphere of TiO2, and other morphology such as nanotube, nanowire, and nanorod. Affecting the photocurrent density (Jsc) and PCE, efficient electron transfer was achieved in HS TiO2. The percentage of PCE of DSSC using HS TiO2 semiconductor is also influenced by physical properties such as particle size and shell thickness, which influence the dye-loading capacity, the rate of electron transfer, and the scattering effects in visible light harvesting. This review discusses the synthesis and characterization of HS TiO2 from two different synthesis pathways, the one-pot and two-step synthesis, and the modification of HS TiO2 to improve the efficiency of DSSC.
Keywords
DSSC
Hollow sphere TiO2
Photovoltaic
Solar cells
TiO2
1. Introduction
The development of renewable energy remains as a great challenge in overcoming the depletion of fossil energy and its negative impact on the environment. Dye-sensitized solar cell (DSSC) is one of the renewable energy devices receiving great attention because it can directly convert sunlight into electrical energy [1]. DSSC is a light and flexible solar cell with simple, low-cost fabrication and can be designed for green devices [2–4]. The main components of DSSC are: a thin layer of semiconductor mesopore, dye molecules as sensitizers, electrolytes, and counter electrodes, which are attached to a conducting glass substrate [4]. A thin layer consists of transparent glass or flexible plastic substrate that can transmit solar radiation into the photoanode. The glass substrate provides a surface for the deposition of semiconductor metal oxides (photoanode) and catalyst material (counter electrode). The photoanode has good electrical conductivity properties to collect the current from the dye-sensitized TiO₂ layer and transfer it to the external circuit [5]. Mesoporous photoanodes are metal oxide semiconductors that play an important role in the loading of dyes and the transfer of electrons to the external circuit [6]. Dye sensitizer (photosensitizer) is a special component in DSSC and is the essence of this type of solar cell. The photosensitizer is attached to the semiconductor through covalent bonds and is responsible for harvesting sunlight, especially in the visible region [7]. Electrolytes are a redox salt solution (e.g. I-/I3-), which is injected between a thin layer of photoanode and the calculating electrode and functions to regenerate the oxidized dye. The last component is the counter electrode (CE), which consists of a thin layer of deposited catalyst. The arrangement of energy levels in all DSSC components must be in harmony to facilitate the transfer of electrons in converting light to electrical energy. Sequentially, the arrangement of energy levels starts from the lowest energy level of the lowest unoccupied molecular orbital (LUMO) of the dye, followed by the conduction band (CB) of the photoanode semiconductor, then the redox potential of the electrolyte, and the energy level of the highest occupied molecular orbital (HOMO) level of the dye [6].
The efficiency of DSSC is influenced by several parameters such as photocurrent (Jsc), photovoltage (Voc), and fill factor (FF) [8–10]. Meanwhile, Jsc relies on the efficiency of light harvesting, injection, and electron collection efficiency [11–13]. According to Ahmad et al. [14], limiting the recombination rate can increase the electron density in the conduction band of the semiconductor as well as increases the quasi-Fermi energy level, and Voc. All of these parameters are closely related to the electron transfer mechanism in the DSSC component. Capturing electrons in a semiconductor is the beginning of photovoltage and the flow of electrons to produce photocurrent [15]. The transfer of electrons in the DSSC component starts from the absorption of photons (hv) by the photosensitizer (dye), which then causes the electron from the dye molecule (D) to experience excitation from the HOMO to the LUMO energy level, as shown in the Eq. (1) [5]:
The excited dye molecule then injects electrons into the conduction band (CB) of the semiconductor, leaving the dye molecule oxidized (D+) (Eq. 2):
The injected electrons are then transmitted through the semiconductor, followed by the transfer of electrical energy through a conductive substrate, external circuit, and a counter electrode (CE, Eq. 3):
Transport of electrons through diffusion process is strongly influenced by the intensity of the incident light, electron concentration, and the efficiency of media transport [5]. At the counter electrode, electrons in the electrolyte component are reduce from I3- to I-. At the same time, the oxidized dye (D+) returns to the ground state (D) after receiving electrons from I- and thus completing the regenerative cycle (Eqs. 4 and 5).
However, in its application, not all electrons can be transferred perfectly in a DSSC device. Electron recombination always accompanies the electron diffusion process in this device. Three types of recombination co-occur with the electron transport process [5,16]. The first is direct recombination; it describes the lifetime of the excited state of the dye molecule. In other words, due to the absorption of sunlight, the excited electrons in the conduction band (CB) return directly to the ground state in the dye molecule’s valence band (VB) (Eq. 6):
Second, recombination in the injection of electrons in the semiconductor (SC) with oxidized dye (Eq. 7):
The third is the recombination of injected electrons in the surface of the semiconductor (SC) with the acceptor in the electrolyte (Eq. 8).
Both reactions in Eqs. (7) and (8) are undesirable as they may result in the losses in the cell efficiency.
Two of the three factors that affect electron transport in the DSSC mentioned above are dye concentration and the efficiency of the electron transport medium [5]. The high dye concentration allows the release of large amounts of electrons from the dye. The efficiency of the electron transport medium is related to the storage capacity and the speed of transferring electrons from the conduction band of the dye molecule to the conduction band of the TiO₂ semiconductor and subsequently to the external circuit [17]. In DSSC, metal oxide semiconductors are photoelectrodes that play a role in electron collection (or electron capture) and transfer, such that their physical properties and structure determine the performance of the DSSC [18]. The structure and physical properties of semiconductors, such as surface area, morphology, and band gap, are some of the factors that affect the efficiency of DSSC [19]. In principle, the efficiency of DSSC depends on the dye loading concentration, and the electron transport speed of the component is strongly influenced by the surface area and pore size of the semiconductor. Whereas the surface area and pore size of the semiconductor are related to the particle size and morphology of the material [20–22].
Various modifications of the semiconductors have been conducted to enhance the efficiency of DSSC. For instance, Ma et al. [23] reported an enhancement in electron transport from the TiO2 double-layer formation. The decrease in particle size results in a blue shift in the band gap of the TiO2 semiconductor. It has an impact on the arrangement of energy levels and electron transfer rates in the device, and the smallest particle size results in the best DSSC efficiency [24]. Examples of the modifications reported in the literature include coating of semiconductor surface with TiOx or TiCl4 solution to reduce the excessive recombination rate [3], incorporating plasmon material as a composite to accelerate electron transfer [25], and modifying the surface morphology in order to increase the surface area to increase the dye loading [26] and their electric properties [22]. A semiconductor with a high specific surface area has a high adsorption capacity. Meanwhile, a large pore volume benefits the electrolyte injection and effective contact between the electrolyte and the photoanode, thereby increasing the photoelectric conversion performance of DSSC [1].
TiO2 nanoparticles with high specific surface area and sufficient dye adsorption capacity are needed to increase the light-harvesting efficiency (LHE) [27]. TiO2 with specific morphology can increase the scattering effect of incident light [26]. TiO2 is a suitable material for use in photoanode applications [17] compared to other metal oxides (such as ZnO, Nb2O5, SnO2, and WO3) because it achieves the highest energy efficiency [6]. TiO2 has wide bandgap, non-toxic, is stable, ecologically benign, cost-effective, and has long-term stability against photo corrosion and chemical corrosion [28–31]. In recent years, research on the morphology of TiO2 as a semiconductor in DSSC reported that hollow sphere HS TiO2 was the best at increasing PCE compared to several other forms, such as nanorods, nanoflowers, and nanotubes. The spherical geometry of these hollow structures facilitates Mie scattering, effectively trapping incident light within the photoanode and prolonging the optical path length. This increased light interaction boosts photon absorption by the dye molecules, thereby enhancing the photocatalytic performance of the DSSC [32]. Moreover, the hollow nature of HS TiO₂ provides a high surface area, offering abundant sites for dye loading [33–36].On the other hand, the hollow structures exhibit good light trapping, charge separation and transport, and surface reactions in photocatalytic applications [37–39]. The improved efficiency in light trapping is attributed to the multiple directions of light scattering and the large surface area photon-retarding effect. Additionally, the hollow structure suppresses the recombination of e- and h+, promoting photocatalytic activity by reducing the charge recombination rate [40], thereby enhancing the light-trapping effect in the photocatalytic process [41]. HS TiO2 with a high specific surface area and sufficient dye adsorption capacity has the potential to enhance electron transfer rates, LHE, and scattering effects to improve DSSC efficiency.
Fabrication of HS TiO2 for the development of DSSC has shown promising advancements in efficiency, as reported by various authors [5,28]. For instance, Tamilselvan and Shanmugan [42] reported an efficiency of 11% using bio-nano materials. Key areas of improvement include molecular engineering or synthesis methods, carrier transport materials, efficient sensitizers, and better electrodes [43,44]. Hydrothermal/solvothermal and sol-gel methods have been widely used to prepare HS TiO2 with various morphology and specific surface area. Exploring efficient HS TiO2 fabricating methods to obtain products with uniform sizes and stable hollow structures is a lengthy process. Until now, there has been a lack of review covering TiO2 HS specifically for DSSC applications. Therefore, the present review discusses the HS TiO2 architecture and explores the synthesis methods, photovoltaic characteristics, and modifications made to improve the efficiency of DSSC. Obtaining precise and significant information about the synthesis pathway and characteristics of HS TiO2 for photoanode applications is the relevant objective of this review.
2. Morphology Effect
A good photoanode is described by a high surface area and light scattering [45,46]. The development of DSSC using commercial TiO2 improves surface area; however, it does not enhance light scattering because the particle diameter is smaller than the wavelength of incident light. Previously, mesoporous multilayer DSSC photoanodes with high surface area and high light scattering capability with an incident photon conversion efficiency of 10% have been developed [47]. Mesoporous materials with pore diameters ranging from 2 to 50 nm possess a continuous network of interconnections, leading to a high surface area and light scattering. Due to these advantages, mesoporous materials serve as a standard for the development of DSSC [48]. However, the mesoporous structure often exhibits low conductivity, which can facilitate the recombination of electrons. Consequently, new nanostructures with high conductivity and enhanced dye loading have become the focus of the next generation of photoanodes [47]. To fulfill the two basic requirements of a good photoanode—high dye loading and light scattering capabilities—the development of TiO2 based photoanodes is still ongoing research. Table 1 compares the different TiO2 morphologies that have been developed for photoanodes with dye loading using commercial dye N719 at the concentration of 5×10-4 M and its photovoltaic parameters.
Morphology | Jsc (mA cm-2) | Voc (mV) | FF (%) | PCE (%) | References |
---|---|---|---|---|---|
Urchin-like | 12.72 | 71.60 | 67.03 | 6.10 | [35] |
Nanowire | 3.60 | 86.80 | 67.60 | 2.12 | [49] |
Nanotubes | 10.48 | 83.50 | 68.12 | 5.96 | [49] |
Nanotubes array | 10.15 | 74.50 | 62.00 | 4.69 | [33] |
Nanorods | 8.88 | 66.00 | 47.00 | 2.75 | [34] |
Hollow nanoparticles | 15.80 | 74.00 | 71.00 | 8.30 | [36] |
Nanoparticles | 4.30 | 69.00 | 72.00 | 2.14 | [50] |
Nanoflower | 5.10 | 79.70 | 72.60 | 2.95 | [51] |
Jsc: short circuit current density, Voc: open circuit voltage, FF: Fill factor, PCE: Power conversion efficiency
Anatase phase TiO2 nanoparticles with a wide band gap are the most effective materials for photoanodes due to their cost efficiency, good stability, easy availability, and compatible optical and electronic properties [22]. TiO2 with different morphologies, such as nanotubes, urchin-like, and hollow nanoparticles exhibiting different PCEs, have been reported (Table 1). It can be seen that nanotubes, urchin-like, and hollow nanoparticles (HNPs) produce high PCE, above 5.0%. The different morphology of TiO2 changes the pore characteristics and the surface area of the material, which also affects the Jsc value. A smaller TiO2 solid particle size can provide a larger surface area to improve the amount of loading of dyes and their Jsc [24,28]. For example, the use of nanotubes (NTs) TiO2 with a surface area of 64.22 m2 g-1 and pore size of 3-10 nm produced dye loading of 103.1 nmol cm-2, Jsc of 10.48 mA cm-2, and PCE of 5.96% [46]. In another study, the urchin-like TiO2 (ULT) that has a higher pore size distribution of 3-60 nm resulted in a higher Jsc of 12.72 mA cm-2 and optimal dye adsorption capacity of about 319.73 nmol cm-2 and PCE of 6.10% [35]. The hollow sphere has been reported to produce the highest PCE than other morphologies; photovoltaic with a Jsc value of 15.8 mA cm-2 and PCE of 8.3% has been produced from HNPs with a surface area of 129 m2 g-1 and particle diameter ∼100 nm [36]. The unique structural properties of HNPs allow for enhanced light-harvesting capabilities and reduced electron recombination, which are critical for improving overall efficiency in DSSCs. As a specific type of HNPs, HS TiO₂ represents an evolution in design that retains these benefits while potentially addressing any limitations in conductivity or charge transport. Therefore, HS TiO₂ is positioned as a promising candidate for further development as a semiconductor in DSSCs, leveraging its excellent properties to maximize efficiency and performance in solar energy applications.
The Jsc depends on the light-harvesting efficiency, injection, and electron collection efficiency mentioned in the early section. Recent studies have demonstrated significant improvements in DSSC performance using hierarchical TiO2 structures. Hierarchical nanostructures are integrated architectures comprising well-ordered nanoscale subunits with zero, one, two, or three-dimensional architectures [52], including nanostructures such as quantum dots, nanofibers, nanorods, nanowires, nanosheets, nanoflowers, nanospheres, or a combination of these architectures [53]. These structures combine the advantages of nanoparticles and larger spheres or rods, offering enhanced dye loading, light scattering, and electron transport [54]. It is reinforced by a hollow structure to improve the surface scattering of light and enhance the incidence of harvesting light [55]. A detailed discussion of the photovoltaic characteristics of hollow spheres TiO2 has been presented in Section 3.
3. Synthesis of HS TiO2
Generally, HS TiO2 can be produced by two synthesis methods: hydrothermal/solvothermal and sol-gel (Figure 1). The hydrothermal/solvothermal method has a single-step synthesis. Meanwhile, the sol-gel method was a two-stage synthesis (Table 2). Synthesis of HS TiO2 with several methods and templates can produce different particle sizes. Hydrothermal/solvothermal methods produce HS TiO2 more than 100 nm in diameter [56], the largest among other methods. The sol-gel (carbon-based) template (SGCT) method produced more than a 50 nm diameter. Meanwhile, HS TiO2, which is produced using a sol-gel hard template (SGHT) method, one of which uses SiO2, can produce a diameter of more than 25 nm [57]. Based on the findings from the previous studies Table 2 presents the diameters of the HS TiO2 produced by the SGHT > SGCT > hydrothermal/solvothermal method.
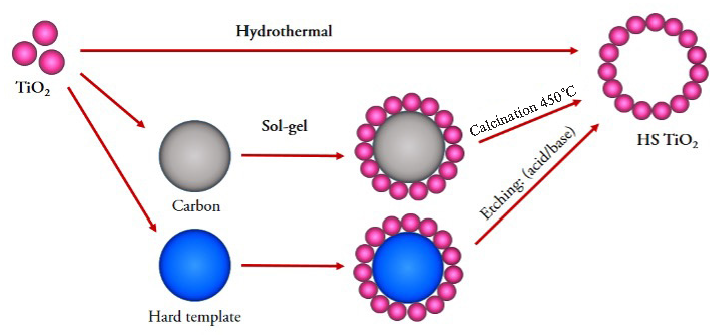
- Schematic of synthesis of HS TiO2.
3.1. Hydrothermal/solvothermal method
Hydrothermal/solvothermal methods were used in many nanoparticle fabrications in the ceramic industry using an aqueous or nonaqueous solution in an autoclave at a high temperature and pressure [58]. In this condition, supercritical fluids are formed and significantly improve the solubility of solid precursors resulting in the formation of nanostructures. In particular, wet fabrication by hydrothermal/solvothermal methods utilizing physical phenomena such as the Kirkendall effect and Ostwald ripening has been widely used to produce hollow nanostructures [59,60].
3.1.1. Kirkendall effect
Synthesis of HS TiO2 by the solvothermal method using ethanol as the solvent at 180°C for 24 hrs resulted in the 1 µm-anatase phase material with a pore size of 5.2 nm and surface area of 242 m2 g-1. Hydrothermal synthesis of HS TiO2 can take place via two formation mechanisms: the Kirkendall effect or Ostwald ripening [61]. Chen et al. [62] reported the production of a pure anatase phase of HS TiO2 with 300–400 nm in diameters and a wall thickness of approximately 50 nm, using polyethyleneimine (PEI), which acts as a stabilizing and templating agent via solvothermal method. Time-dependent morphological evolution using transmission electron microscopy (TEM) analysis confirmed the formation of HS TiO2 via the Kirkendall effect (Figure 2). In the synthesis of HS TiO2 through the Kirkendall effect, solid nanospheres are formed at the beginning of the reaction (Figure 2a) (before heating). After heating at 180°C for 6 hrs, the nanosphere size and surface roughness increased, and a small cavity began to form (Figure 2b). When the reaction time increased to 16 hrs, the increase in the nanosphere particle size and inner cavity size was observed (Figure 2c). Meanwhile, different quantity of PEI was associated with the different viscosity of the reaction system and the adsorption of PEI molecules on the TiO2 surface and these controlled the morphology of the TiO2 produced [62]. Chen et al. [62] and Zhu et al. [63] reported that calcination treatment after the completion of the reaction at 400 to 700°C further enhanced the crystallinity of the TiO2 without changing the crystalline phase.
![TEM images (a-c): the formation of hollow TiO2 at different reaction times of 0, 6, and 16 h. (d) Scheme illustration of the formation of a TiO2 hollow nanosphere via the Kirkendall effect [62]. Licensed by Elsevier.](/content/184/2025/18/3/img/AJC-18-1272024-g10.png)
- TEM images (a-c): the formation of hollow TiO2 at different reaction times of 0, 6, and 16 h. (d) Scheme illustration of the formation of a TiO2 hollow nanosphere via the Kirkendall effect [62]. Licensed by Elsevier.
The Kirkendall effect basically refers to the process of nonequilibrium mutual diffusion through the atoms in a diffusion couple. This causes supersaturation of the vacancies at the interface, and further condensation of the excess vacancies and interdiffusion leads to the formation of a hollow sphere [64,65]. The Kirkendall effect has been used in the fabrication route to form HS. However, not every particle size, shell thickness, or material crystallinity can be obtained from this route [66]. Basically, synthesis through the Kirkendall effect requires a surfactant to produce HS TiO2. The Kirkendall effect is a one-pot synthesis with a two-step process that can produce HS TiO2. The first stage is the formation of vacancies in the TiO2 nanostructure. In this stage, the faster-diffusing species will be confined into a nanocrystal core. The vacancy-injection net rate increased, as evidenced by the high surface-to-volume ratio of the transformed particles and the absence of defects in the TiO2 nanostructure. In the next stage, supersaturated void clouds will gather together and form a cavity of HS TiO2 (Figure 2d) [67].
Method | Materials | Synthesis condition | Characteristics | References | |||||
---|---|---|---|---|---|---|---|---|---|
Template/surfactant | Precursor | Temperature (°C) | Time (hour) | Surface area (m2/g) | Particle size (nm) | Pore size (nm) | Wall thickness (nm) | ||
Hydrothermal/solvothermal | Urea, tert-Butyl ammonium hydroxide | Titanium isopropoxide | 180 | 24 | 78.3 | 3000 | [68] | ||
Tween 60 & polyethylene glycol | Titanium isopropoxide | 180 | 24 | 155 | 1000 | [69] | |||
Oxalic acid | Titanium n-butoxide | 180 | 10 | 450 | 30 | [70] | |||
Polyethyleneimine | Tetrabutyl titanate | 180 | 24 | 100-200 | 2-50 | [63] | |||
Glucose, polyvinyl pyrrolidone (PVP) | Titanium tetrabutoxide (TBOT) | 125 | 72 | 75-100 | 10-14 | [71] | |||
Valeric acid, butyric acid | Titanium isopropoxide | 190 | 18 | 83 | 514 | 7 | [72] | ||
NH4F, CO(NH2)2 | Ti(SO4)2 & Zn(NO3)2·6H2O | 180 | 6 | 55.985 | ∼2000 | [73] | |||
Octanoic acid | Titanium isopropoxide | 190 | 18 | 47.5, 52.1, 55.9, 54.0 | 220, 440, 620, 800 | [55] | |||
Polyethyleneimine (PEI) | Butyl titanate | 180 | 24 | 300-400 | 50 | [62] | |||
Tween 20 | Titanium isopropoxide | 180 | 24 | 242 | 52 | [74] | |||
Acetylacetone | Tetrabutyl titanate | 200 | 10 | 900 | ∼700 | ∼90 | [75] | ||
Urea | Ti plate: TiCl4:H2SO4 | 200 | 12 | 1000 | 20-30 | [76] | |||
Titanium(IV) butoxide (TBT) | 220 | 5 | 1000-2000 | [56] | |||||
Tetrabutyl titanate | 130 | 48 | 10 | 2-4 | [67] | ||||
Oxalic acid | Tetrabutyl titanate | 180 | 6 | 166.2 | 1000-1500 | 3-33 | 150 | [77] | |
PVP, NaF | Tetrabutyl titanate | 110 | 2, 3, 4, 5 | 320 | [78] | ||||
H3BO3 | AHFT: (NH4)2TiF6 | 90 | 0.25 | 102 | 800-1000 | [79] | |||
Titanium (IV) chloride | 220 | 6 | 50.2 | 1000 | 18.7 | [80] | |||
Tetrabutyl titanate (TBOT) | 220 | 5 | 1200 | 100 | [81] | ||||
Sol-gel (carbon-based template) | Polystyrene | TTIP | 50 | 1.5 | ∼380, ∼190 | ∼60, ∼50, ∼100 | [82] | ||
Polystyrene | Tetra-n-butyl titanate (TBT) | RT | 0.5 | 41, 36, 34 | 17, 21, 18 | [83] | |||
Polystyrene | Titanium tetrabutoxide (TBOT) | 80 | 2 | 147, 151, 155, 159 | 9, 14, 17, 23 | [84] | |||
Polystyrene | TBOT | 70 | 2 | 96.7, 54.2 | 332, 412 | 11.3, 8.1 | 28, 50 | [85] | |
Polystyrene | Titanium ethoxide | 70 | 12 | 60-365 | 100, 110, 120, 130 | [86] | |||
Poly styrenemethacrylic acid (PSA) | Ti(SO4)2 | 50 | 1.5 | 20.821, 52.412, 113.335 | 1000-120 | [87] | |||
Carbon hollow sphere | Titanium(IV) n-butoxide (Ti(OBu)4) | 24.85 | 24 | 400-600 | 20-60 | [88] | |||
Glucose | Titanium tetraisopropoxide (TTIP) | 1 | 250-350 | [89] | |||||
Polystyrene | tetrabutyl titanate (TBT), tetrabutylzirconate (TBZ) (1:1) | 25 | 3 | 190 | [84] | ||||
Polystyrene | tetrabutyl titanate (TBT) | 80 | 6 | 58.3 | 300 |
13.3 |
[90] | ||
CTAB | TiCl4, (CH3CH3CHO)4Ti | 2 | 94.9 | 36000 | [57] | ||||
Polydopamine (PDA) | Tetrabutyl titanate (TBT) | RT | 2 | 568 | 105 | [91] | |||
Anhydrous dextrose | Titanium (IV) butoxide | RT | 1 | 138.07, 101.98, 54.53 | 667.98, 327.03, 170.91 | 46.98, 28.36, 32.62 | [92] | ||
Polystyrene | Tetrabutyl titanate (TBT) | RT | 20 | 85 | 59 | 16 | [93] | ||
Sol-gel (hard template) | SiO2: (TEOS) | Titanium tetraisopropoxide (TTIP) | 55, 50, 40, 35, 20 | 6 | 25, 50, 75, 100, 125 | [94] | |||
SiO2: (TEOS) | Titanium tetraisopropoxide (TTIP) | 50 | [95] | ||||||
SiO2: (TEOS) | Titanium tetraisopropoxide (TTIP) | 5 | 6 | 25, 50, 100 | 5.5, 11, 20.5 | [96] | |||
SiO2: (TEOS) | Titanium n-butoxide (TBOT) | 85 | 2 | 360 | Outer: 30, Inner: 35 | [97] | |||
SiO2: (TEOS) | Titanium tetraisopropoxide (TTIP) | 4 | 6 | 246 | 120 | [98] | |||
Au: HAuCl4 | TiF4 | 100 | 1 | 200 | 60 | [99] | |||
SiO2: (TEOS) | Titanium tetraisopropoxide (TTIP) | 4 | 6 | 129 | ∼100 | Cav: 5, Intra: 35 | [36] | ||
K3PW12O40 | Ti(SO4)2 | 125 | 8 | 500-1000 | [100] | ||||
CaCO3 | TEOS, TBOT | RT | 3 | 113 | <181 | [101] | |||
Au: HAuCl4 | TiF4 | 180 | 1 | ∼200 | [102] | ||||
SiO2: (TEOS) | tetrabutyl titanate (TBT) | 85 | 1.7 | ∼200 | ∼50 | [103] | |||
SiO2: (TEOS) | Titanium tetraisopropoxide (TTIP) | 12 | 140 | 20 | [104] | ||||
SiO2: (TEOS) | Titanium n-butoxide (TBOT) | 2 | 185 | 30 | [105] | ||||
SiO2: (TEOS) | Titanium n-butoxide (TBOT) | RT | 2 | 455, 470, 494, 530, 606 | 23, 31, 41, 61, 99 | [106] | |||
SiO2: (TEOS) | Titanium Isopropanol | 25 | 1.5 | 74.3 | 204 | 17 | [107] | ||
SiO2: (TEOS) | Titanium n-butoxide (TBOT) | 45 | 24 | 480 | 20-30 | [108] |
RT: Room temperature, PVP: Polyvinylpyrolidone, NaF: Sodium flouride, TTIP: Titanium isopropoxide, CTAB: Cetyltrimethylammonium Bromide
3.1.2. Ostwald ripening
The formation of HS TiO2 through Ostwald ripening is a one-pot synthesis without a template, surfactant, and calcination process. This process is a growth from a solid bulk structure, spherical solid structure to a spherical hollow structure [90]. High pressure can affect colloidal crystallite suspensions, causing compression and potentially altering electrostatic properties while also increasing orientational order and crystallite growth [109]. Ostwald ripening is also described as dissolving the core and recrystallizing the shell, resulting in a cavity of the structure (Figure 3) [70].
![TEM images of the samples with different reaction times: (a) 3 h at 150°C. If not stated, all the reactions are at 180°C. (b) 2 h; (c) 3 h; (d) 4 h; (e) 5 h; (f) 6 h; [70]. Licensed by American Chemical Society. TEM: Transmission electron microscopy.](/content/184/2025/18/3/img/AJC-18-1272024-g11.png)
- TEM images of the samples with different reaction times: (a) 3 h at 150°C. If not stated, all the reactions are at 180°C. (b) 2 h; (c) 3 h; (d) 4 h; (e) 5 h; (f) 6 h; [70]. Licensed by American Chemical Society. TEM: Transmission electron microscopy.
The production of HS TiO2 through Ostwald ripening begins with the etherification of alcohol as a solvent by H2O. The TiO2 precursor undergoes hydrolysis and forms a cavity in the hollow structure (Figure 4). Synthesis with this principle is very dependent on the reaction temperature. At temperatures less than 140°C, alcohol etherification is difficult, and hydrolysis of TiO2 precursors cannot be carried out, so HS TiO2 is not formed. When the temperature exceeds 140°C, the system allows for the formation of HS TiO2 from the precursor, but the reaction rate will vary at different temperatures [90]. In a reaction rate investigation, Nguyen and Kim [110] reported that HS TiO2 was successfully produced through Ostwald ripening at 220°C. HS TiO2 with anatase crystal phase was formed after the reaction was running for 5 h, and the crystallinity and structure after being extended 7 hrs did not change. Meanwhile, control of the diameter of HS TiO2 can be done by adjusting the addition of Ti precursors, but this can impact decreasing the pore diameter and surface area [56]. Excess precursors are also thought to reduce the core’s dissolution process in cavity formation.
![Scheme to illustrate the formation process for hollow TiO2 spheres [90]. Licensed by Elsevier.](/content/184/2025/18/3/img/AJC-18-1272024-g12.png)
- Scheme to illustrate the formation process for hollow TiO2 spheres [90]. Licensed by Elsevier.
3.2. Sol-gel method
3.2.1. Sol-gel carbon-based template
Fabrication of HS TiO2 by the sol-gel method has a two-step synthesis. Templating the HS TiO2 by producing a core@shell structure is the first step, and removing the templates (core) to produce a hollow sphere is the second step. The sol-gel method uses two templates to get core@shell, carbon-based, and hard templates. Polydopamine (PDA) has also shown good performance as a carbon-based template (CBT) in the manufacture of HS TiO2. The synthesis of HS TiO2 with a PDA template was reported by Ma et al. [91], resulting in HS TiO2 with a diameter of ∼500 nm for photocatalytic applications.
In principle, PDAs have demonstrated the ability to effectively adhere to many surface materials such as metals, oxides, ceramics, polymers, carbon nanotubes, and magnetite nanoparticles [111]. The other CBT used to produce HS TiO2 from the sol-gel process include poly(styrenemethacrylic acid) (PSA) [87], glucose [89], and polystyrene (PS) [83]. As a template, PS is produced by the polymerization of styrene. To be able to deposit TiO2 on the PS surface, it is necessary to add a cationic initiator such as 2-(methacryloyloxy)ehyl trimethylammonium chloride (DMC) (Figure 5) [112]. Similarly, cationic polymerization initiators like 2,2-azobis-(isobutyramidine) dihydrochloride (AIBA) [85] and potassium persulfate (KPS) [84] have been reported to provide positive charges on the surface of PS and assist the formation of PS@TiO2 (Figure 5). The formation of HS TiO2 with different particle sizes can be affected by pH and the ratio of the addition of titania precursor to solvent. For example, Kanjana et al. [92] reported that a reaction at low pH (acidic) resulted in larger particle size compared to reactions conducted under neutral or alkaline conditions. In addition to that, Syoufian et al. [84] reported that adding an excessive amount of precursor increased the particle size of HS TiO2. The TiO2 template removal to produce the hollow structure can be carried out simultaneously with calcination. Synthesis with this method can also produce HS TiO2 at low pressures to yield particle sizes of hundreds to thousands of nm and shell thicknesses of up to hundreds of nm (Table 2).
![Synthetic route of PS/TiO2 core@shell particles [112]. Licensed by Elsevier.](/content/184/2025/18/3/img/AJC-18-1272024-g13.png)
- Synthetic route of PS/TiO2 core@shell particles [112]. Licensed by Elsevier.
3.2.2. Sol-gel hard template
The sol-gel hard template method is a two-step process commonly used to synthesize hollow nanostructures like HS TiO₂ [113]. The first stage is templating core@shell with SiO2 as the core coated with TiO2 in the shell. The second stage is the formation of HS TiO2 by removing the core from the core@shell (Figure 6). The synthesis of HS TiO2 through the formation of SiO2@TiO2 was carried out at alkaline pH to prepare a SiO2 template with a negative surface charge (-) to interact with the titania precursor prepared in acidic medium (positive surface charge). The synthesis of HS TiO2 can be conducted by SiO2 core from SiO2@TiO2 material using ammonia or sodium hydroxide solution by etching [95–97, 107]. The fabrication process involves the introduction of ammonium ions (NH₄⁺), which bind to the negatively charged SiO₂ surface through electrostatic interactions with the –OH groups, creating a positively charged surface. This step ensures strong adhesion of the titania precursor to the SiO₂ template, enabling the formation of a uniform TiO₂ shell.
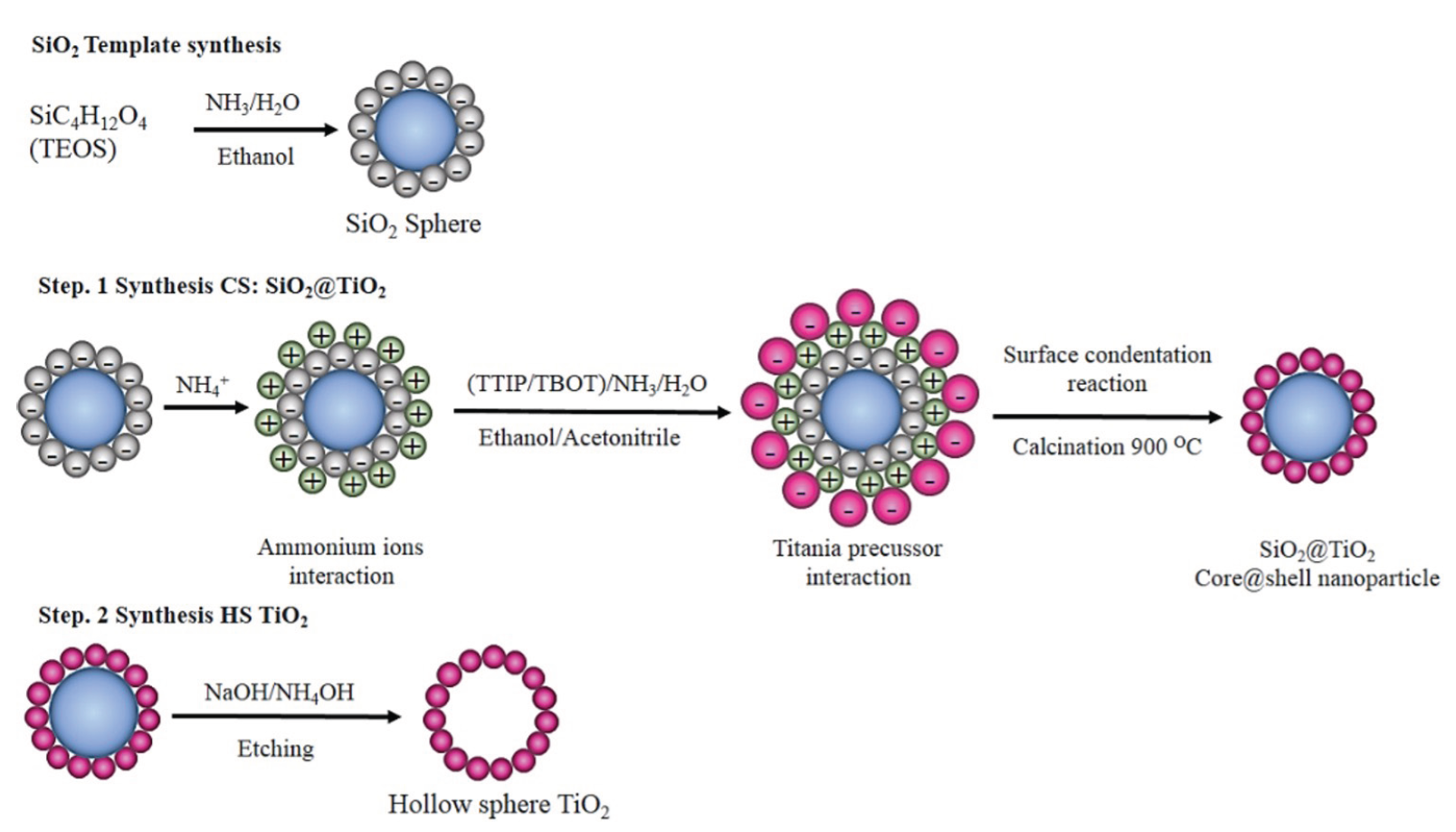
- Schematic of two step HS TiO2 synthesis via SiO2@TiO2.
The accumulation of NH4+ on the surface of SiO2 encourages interaction with the negatively charged –TiOH group and, through a condensation process with an NH4+ catalyst forms SiO2@TiO2. The formation of a hollow material can be determined by core@shell (CS) as the initial product of HS TiO2 synthesis. In manufacturing the SiO2@TiO2, SiO2 is widely used as a core because it is easy and fast to prepare. The Stöber method is a well-known successful method for producing monodisperse SiO2 spheres [114]. Moreover, SiO2 has good solubility in based solution, making this compound easy to remove. In the final step, ammonia or sodium hydroxide solution was used to remove the SiO2 core from SiO2@TiO2 by etching [95–97, 107]. Adding a hard alkaline solution to SiO2@TiO2 through sonication and stirring pushes high-intensity energy into the voids between the silica-titania, which causes partial etching of the silica core. Under conditions of strict induction reaction, it caused corrosion and etched silica-titania part. The etched silica and titania species are released into the solution and diffused from the nanosphere to the titania shell. Furthermore, the etched fragments undergo condensation and re-deposition on the titania shell’s surface through Ostwald maturation (reverse reaction). This reversible reaction continues until a hollow nanosphere structure is formed [103]. The anatase phase of HS TiO2 was obtained after calcination of SiO2@TiO2 at 500-900°C [97,103,105]. Calcination at 900°C showed the optimal temperature of the anatase phase of HS TiO2 [103]. At high temperatures, the slowing formation of the crystal structure of anatase HS TiO2 occurs due to interaction with SiO2 as a core [103].
Hollow sphere TiO2 can also be synthesized with a hard template using gold (Au) from HAuCl and several inorganic salts (Table 2). The manufacture of HS TiO2 with an Au template began with the formation of Au@TiO2 resulting from the sol-gel method at more than 100°C. The HS TiO2 was obtained by depositing the Au core with KCN [99]. The fabrication of HS TiO2 has also been reported by Chen et al. [101] which uses the CaCO3 template through a two-step reaction. The hollow sphere formation was obtained after etching CaCO3 using HCl [101]. Lan et al. [100] reported the use of K3PW12O40, obtained by mixing a solution of KCl and H3PW12O40, as a template to produce HS TiO2 with a diameter of 0.5-1 µm. The template was removed using 1 M NaOH solution [100]. Rakhman et al. [115] introduced a novel two-step synthesis method to fabricate HS TiO₂, achieving optimal crystallite sizes of 9.53 to 20.54 nm and micro-strains. The resulting hollow spheres demonstrated excellent photooxidative properties under UV irradiation, highlighting their potential for solar cell applications. Similarly, a one-pot sol-gel method has been applied for synthesizing HS TiO₂ embedded with silver nanoparticles (AgNPs), achieving uniform distribution of AgNPs on the HS TiO₂ and enhanced light harvesting for DSSCs [116].
Several existing synthesis methods have similar techniques for producing HS TiO2 with different particle sizes. In the hydrothermal carbon-based templates and hard templates method, adding excess titania precursors was adopted to design HS TiO2 with larger particle sizes. However, in the hydrothermal/solvothermal method, this approach will affect the cavity size of the HS TiO2. The sol-gel method using a template can improve the shell’s thickness in this situation. The template-assisted method also makes it possible to design the size of the HS TiO2 cavity. Kanjana et al. [92] reported that the formation of HS TiO2 with different particle sizes could be influenced by the pH and ratio of addition of the titania precursor to the solvent. The reaction at low pH (acidic) gave a larger particle size compared to neutral or alkaline conditions in the sol-gel carbon-based template synthesis [117].
In addition, other methods like co-precipitation and solid-state methods can also be used to synthesize HS TiO2 [81,118]. However, this review focuses on the hydrothermal/solvothermal and sol-gel methods, as both these synthesis routes are the most popular among all.
3.3. Characterization of HS TiO2
Scanning electron microscopy (SEM) and transmission electron microscopy (TEM) images are the primary data to determine the morphology of HS TiO2. The data such as diameter size, particle size, grain size, crystal size, cavity, and shell thickness can also be obtained using both. Other data, such as binding energy curve by x-ray photoelectron spectroscopy (XPS), elemental mapping plot by energy dispersive X-ray spectroscopy (EDS), and absorption spectra by IR, were added by several researchers to provide information on reaction products at each stage, especially in the sol-gel method using templates. Interestingly, several articles discussed changes in the structure of the TiO2 products to show the progress of the reaction. For example, Chen et al. [62] showed TEM images emphasizing the Kirkendall effect process (Figure 2) in producing HS TiO2. Zhao et al. [119] studied the reaction rate of HS TiO2 formation through Ostwald ripening (Figure 3) with TEM images.
Most of the characterization of HS TiO2 by SEM and TEM through two-stage sol-gel synthesis presented different images of templates, intermediate materials, and hollow sphere products. In the carbon-based templates method, Song and Gao [85] presented a TEM images (Figure 7) of polystyrene (PS) sphere, PS@TiO2, and HS SiO2/TiO2.
![TEM images: (a) polystyrene (PS) spheres, (b) PS@TiO2, (c) HS SiO2/TiO2 [85]. Licensed by American Chemical Society. TEM: Transmission electron microscopy.](/content/184/2025/18/3/img/AJC-18-1272024-g15.png)
- TEM images: (a) polystyrene (PS) spheres, (b) PS@TiO2, (c) HS SiO2/TiO2 [85]. Licensed by American Chemical Society. TEM: Transmission electron microscopy.
The PS ball template (Figure 7a) looks clear and smooth with a uniform size. The TiO2 coating forming PS@TiO2 (Figure 7b) shows a different image of the PS sphere with a rougher surface and larger particle size changes. Meanwhile, the HS SiO2/TiO2 formed after the removal of the PS core shows a smoother ring image than PS@TiO2 (Figure 7c). Karabacak et al. [120] show the PS sphere’s change to PS@TiO2 with the SEM image (Figure 8). Similar to the TEM image, the SEM image shows a smoother PS sphere surface of uniform size (Figure 8a) compared to PS@TiO2, which offers a rougher surface (Figure 8b).
![SEM images: (a) Polystyrene, (b) PS@TiO2 [120]. Licensed by Elsevier.](/content/184/2025/18/3/img/AJC-18-1272024-g16.png)
- SEM images: (a) Polystyrene, (b) PS@TiO2 [120]. Licensed by Elsevier.
The formation of PS@TiO2 as an intermediate for HS TiO2 through a sol-gel pathway based on a carbon template was supported by IR spectroscopy. Shi et al. [121] confirmed the formation of PS@TiO2 by comparing the IR spectra of PS, PS@TiO2, and TiO2 anatase. The difference in absorption bands of the three indicates the interaction of PS and TiO2. The PS microspheres showed absorption bands around 3000 and 1470 cm-1, representing the C-H and C-C stretchings of the phenyl group [121]. Absorption around 760 and 700 cm-1 was associated with bending vibrations of phenyl in the C-H and C-C groups. While the absorption at 650 cm-1 is related to solid Ti-O-Ti vibrations [121]. The production of HS TiO2 by the sol-gel synthesis pathway using SiO2 as the template was also confirmed via TEM images (Figure 9). The different solid structure of SiO2@TiO2 (Figure 9a) with ring-like HS TiO2 (Figure 9b) followed by a change in the percentage of silica and titania in the EDS spectra indicated the success of dissolving the SiO2 core by NaOH [36].
![TEM images and EDS spectra (inset) of (a) SiO2@TiO2, (b) HS TiO2 [36]. Licensed by American Chemical Society. TEM: Transmission electron microscopy.](/content/184/2025/18/3/img/AJC-18-1272024-g17.png)
- TEM images and EDS spectra (inset) of (a) SiO2@TiO2, (b) HS TiO2 [36]. Licensed by American Chemical Society. TEM: Transmission electron microscopy.
In addition to the primary data from TEM and SEM images, the formation of SiO2@TiO2 in several articles is shown by supporting data such as absorption spectra by fourier tranform infrared spectroscopy (FTIR), binding energy curve by XPS, and qualitative data on elemental percent by EDX (Table 3). The FTIR spectra specifically showed Si-O-Ti interaction on the formation of SiO2@TiO2 in the absorption area of 940-962 cm-1. While the analysis of bond energy (B.E) with XPS on O1s measurements showed 2 to 3 peaks, with the characteristics of Ti-O-Ti at 527.6-530.2 eV, Ti-O-Si at 530.7-532.5 eV, and Si-O-Si at 531.5-533.3 eV. The SiO2@TiO2 characteristic is also shown in the reflectance and absorbance of UV/Vis spectra (Figure 5). The reflective index (n) of SiO2@TiO2 was between SiO2 (1.47) dan TiO2 (2.49) nanoparticles at 400-700 nm. Meanwhile, the UV/Vis SiO2@TiO2 absorption spectra showed higher adsorption than SiO2 and TiO2 nanoparticles at 400-650 nm [122].
4. Photovoltaic Characteristics
4.1. Scattering light ability of HS TiO2
TEM image showing HS TiO2 appears as a ring, indicating the presence of a cavity. It is a unique feature of HS TiO2 in addition to the pores of the interface (intra-shell). This feature allows a strong internal light scattering in the HS TiO2 compared to solid particles [98]. Lei et al. [123] investigated the light scattering properties of HS TiO2 as indicated by the UV-Vis reflectance spectrum (Figure 10a). HS TiO2 exhibits strong reflectance in the 400-800 nm range to more than 50%, while the TiO2P25 is lower.
In addition, HS TiO2 also shows a stronger visible light absorption ability than TiO2 P25 [69]. It is attributed to the ability of the hollow structure to absorb more photons than TiO2 P25 [124]. The dye loading on HS TiO2 also showed a higher amount than TiO2 P25. However, dye-sensitized TiO2 HS was able to maintain a higher reflectance than TiO2 P25 [77]. This condition is associated with a greater opportunity for the dye to absorb photons [26]. The multi-reflection effect arises from HS TiO2 to scatter incident light at different wavelengths in the visible area, and the scattering effect can increase the optical path of the incident light (Figure 10b). This condition contributes to higher light-harvesting efficiency to increase photocurrent density (Jsc) [123].
4.2. Photovoltaic performance
As mentioned earlier, the unique feature of HS TiO2 is a distinct advantage in photovoltaic characteristics. Lee et al. [125] reported that the structural effect of HS TiO2 was important, where HS TiO2 showed better light absorption compared to the same particles after crushing into powders with a size of 10 nm. Despite having the same band gap at 3.2 eV as anatase TiO2, the diameter and thickness of the HS TiO2 shell were different, indicating different visible light absorption activities in the 400-800 nm area. This condition confirms that the morphology of HS TiO2 has a different effect on its photoactivity [106]. The HS TiO2 with a diameter of ∼100 nm provided the best PCE [36]. In addition to that, the diameter of the HS TiO2 also affects the dye loading ability (Figure 11), where a larger HS TiO2 diameter caused a reduction in the amount of dye loading [138]. This condition is thought to occur when light incident on the surface of the material experiences an extension of the wavelength path in the cavity, enhancing photon capture by dye molecules [36,99]. The large surface area of particles allows the dye loading to be higher than the solid particles, thus encouraging more optimal photon harvesting [98].
FTIR | XPS B.E. (eV) | SEM/FESEM/TEM/HRTEM | EDX | References | ||||||||
---|---|---|---|---|---|---|---|---|---|---|---|---|
Ti-O-Si | Si 2p | O 1s | Ti 2p |
Particle size (nm) |
Crystallite size (nm) |
Core size (nm) |
Shell thickness (nm) |
Si:Ti (%) | ||||
(cm-1) | Si-O-Si | Ti-O-Ti | Ti-O-Si | Si-O-Si | Ti 2p1/2 | Ti 2p3/2 | ||||||
940 | 529.9 | 532.5 | 533 | ∼440 -∼560 | [126] | |||||||
40 | [114] | |||||||||||
110, 240, 530 | 46:54, 80:20, 92:8 | [122] | ||||||||||
200, 260, 300, 320, 370 | [127] | |||||||||||
81.2, 101.6, 124.4, 152.5 | 55.54:44.46 | [128] | ||||||||||
101.8 | 531.5 | ∼130 | [129] | |||||||||
225, 245, 250, 265 | 4.4, 4.5, 4.3, 4.6 | 200 | 23, 25, 34, 33 | [130] | ||||||||
962 | 530.1 | 531.9 | 532.9 | 464.4 | 458.8 | 14.85 | ∼310 | [131] | ||||
943 | 530.2 | 531.9 | 532.6 | 464.2 | 458.6 | 450-550 | ∼310 | [132] | ||||
402 | ∼7 | 38.60:1.15, 42.09:7.53, 25.29:14.49 | [133] | |||||||||
102.7 | 464.2 | 458.8 | 390 | 65 | [23] | |||||||
955 | 18.18, 18.26, 22.75, 28.98, 38.18 | 221 | 7, 17, 25 | 36.6:0.92, 36.02:1.56, 28.22:2.91 | [134] | |||||||
101.41 | 527.63 | 530.73 | 462.13 | 456.18 | [135] | |||||||
104.2 | 530.2 | 531.6 | 533.3 | 222 | 193 | [136] | ||||||
103.9 | 533 | 464.1 | 458.4 | 100 | 0.35 | [137] |
The higher photon absorption of HS TiO2 is indicated by a decrease in wavelength (350-800 nm) in the transmittance spectrum [99]. This phenomenon is associated with the efficiency of current conduction (electron transfer) from the dye by HS TiO2 to the DSSC system [26]. This indication is seen in the pore characteristics and surface area of the material that affect the loading ability of the dye molecules and the electron transfer speed of the dye molecules [26].
4.3. Enhancing photovoltaic characteristic
Modifications of HS TiO2 as a semiconductor component in DSSC have been reported to enhance the Jsc and PCE (Table 4). It is reported that the combination of HS TiO2 and TiO2 nanosheets enhanced Jsc by 2.44% and PCE by 0.96% [119]. Meanwhile, HS TiO2 composite films with TiO2 nanotube arrays enhanced Jsc by 3.05%, with a 1.31% improvement in PCE [140]. Adding non-semiconductor materials such as carbon nanotubes enhanced Jsc by 1.6% and PCE by 0.77% [141]. Meanwhile, 0.25 mol% Fe doping on HS TiO2 increased Jsc by 4.84% and PCE by 2.39% at the optimum conditions, which have the surface area and dye loading of 122.84 m2 g-1 and 22.12×10-8 mol cm-2, respectively [142]. Additionally, a study reported that DSSCs fabricated with Fe/S–TiO₂ photoanodes exhibited an improved solar-to-electrical energy conversion efficiency of 6.46%, compared to 3.43% for pure TiO₂, under full sunlight illumination [143].
Modifications | Enhancement of Jsc (%) | Enhancement of Voc (%) | Enhancement of PCE (%) | References |
---|---|---|---|---|
Film composite HS TiO2/carbon nanotubes | 1.6 | 0.013 | 0.77 | [141] |
Double light-scattering layer HS TiO2 and nanosheet TiO2 | 2.44 | 0 | 0.96 | [119] |
TiO2 HS/TiO2 nanotube array composite films | 3.05 | -0.01 | 1.31 | [140] |
TiO2 HS assembly from nanosheets | 3.99 | -0.01 | 2.22 | [30] |
Fe-doped TiO2 HS | 4.845 | 0.038 | 2.39 | [142] |
HS TiO2 decorated small Ag NP | 3.1 | 0 | 1.2 | [98] |
HS TiO2 decorated large Ag NP | 4.3 | 0 | 1.9 | |
HS TiO2 decorated Ag NP | 1 | 0.01 | 0.7 | [36] |
HS TiO2 decorated Au NP | 0.5 | 0.01 | 0.5 | |
HS TiO2 decorated Au@Ag NP | 1.5 | 0.01 | 1.1 |
Another modification is the addition of localized surface plasmon resonance material (LSPRM), such as Au, Ag, or alloys of both, which increased the PCE by 0.5, 0.7, and 1.1 %, respectively [141]. The addition of Ag with a larger particle size gave an even higher PCE of 1.9 % [98]. According to Song et al. [144], LSPRM in the DSSC system increased the number of electrons released from the dye and the electron density of the semiconductor. The interfacial interaction between LSPRM and the semiconductor causes a fast electron transfer from the dye molecule [145]. The plasmon effect can be formed when the light comes in and stimulates free electron oscillations in LSPRM [146]. When the LSPRM on the surface of the semiconductor material is excited by light, a strong electromagnetic field is generated. A strong electromagnetic field can enhance the photocurrent and regeneration of photoelectrons [147]. However, the addition of a higher concentration of LSPRM could attenuate the efficiency of the DSSC due to a decrease in dye loading on the TiO2 semiconductor [146]. Enhancing the local electromagnetic field around the nanostructure is the key to increasing dye adsorption in the DSSC system [148]. LSPRM plays multiple roles in the performance of DSSCs, including an enhancement in the dye adsorption coefficient and optical absorption due to surface plasmonic resonance [149]. LSPRM also acts as an electron sink for charge carriers on photoinduction, enhancing the interfacial electron transfer process and minimizing electron transfer recombination, thereby enhancing the electron transfer process in DSSC [148].
HS TiO2 has demonstrated excellent photovoltaic performance under laboratory conditions. However, several challenges might hinder its practical applications. One significant challenge is the stability and durability of HS TiO₂ structures. Over time, environmental factors such as moisture, UV radiation, and temperature fluctuations can lead to degradation, resulting in a decline in photovoltaic performance. Implementing protective coatings or encapsulation techniques can shield HS TiO₂ structures from these environmental stressors [150]. Manufacturing scalability is another concern. The synthesis of HS TiO₂ structures often involves complex processes that may not be easily scalable for large-scale production [151]. Therefore, scaled-up productions of HS TiO2 in order to establish the best performance and cost-effective synthesis route is required.
The addition of LSPRM to HS TiO2 provides an opportunity to enhance the efficiency of the DSSC compared to the single semiconductor material in the photoanode. It provides a better prospect for DSSC development [36]. Thus, it raises several new challenges to conduct morphological tracing and suitable LSPRM arrangement on HS TiO2-based photoanode components to increase the work conversion efficiency (PCE) of DSSC.
5. Conclusions and Outlooks
Developing HS TiO2 as a semiconductor material for DSSC components is important for improving the efficiency of DSSC. HS TiO2 offers significant morphological advantages, which can improve the PCE of DSSC. The unique morphology of HS TiO2 contributes to improved short-circuit current density (Jsc) and, consequently, a higher PCE. This review highlights the synthesis of HS TiO2 and its photovoltaic properties as key areas of innovation.
This review presents several HS TiO2 synthesis methods, such as hydrothermal/solvothermal, sol-gel by carbon-based template, and hard template. Hydrothermal/solvothermal has the advantages over these methods because it has the shortest synthesis route compared to the sol-gel method for the formation of the HS TiO2 structure as well as the arrangement of the crystal phase. Meanwhile, the fabrication of HS TiO2 through the hard template sol-gel method can provide a smaller pore size than other synthesis pathways. The uniqueness of HS TiO2 pores, smaller particle size, and large surface area allow the acceleration of electron transfer from the dye to the device and show a higher Jsc than other morphologies. Although the HS TiO2 from the SiO2@TiO2 produced smaller particle sizes and higher PCE compared to other pathways, the synthesis of this pathway requires the longest fabrication time starting from the formation of SiO2@TiO2, then the growth of the crystal phase by calcination, and followed by the removal of the SiO2 core through etching.
Hollow sphere TiO2 can scatter light very well due to the influence of its micropore particles and the uniqueness of its cavity. This property is the advantage of HS TiO2 for photocatalyst applications and solar cells. Especially in DSSC applications, semiconductor HS TiO2 has several challenges, including the loading dye capacity and the electron transfer rate, which still depend on the particle size of the HS TiO2. The particle size of HS TiO2 is one of the variables studied for its ability as a dye adsorbent, incident light scattering properties, and electron transfer speed in DSSC applications. So far, the thickness of the HS TiO2 shell has not received similar attention in these studies. According to Mie’s theory, the thickness of the HS TiO2 shell can affect the scattering of incident light. In the future, therefore, the thickness of the HS TiO2 shell would be the other key parameter affecting the conversion of light into electricity in DSSC.
Recent advancements in DSSCs have focused on enhancing the dye loading and light-harvesting efficiency through various material modifications. Incorporating carbon nanotubes (CNTs) into the photoanode has been shown to improve electron transport and reduce recombination rates. For instance, research demonstrated that integrating multi-walled carbon nanotubes (MWCNTs) into the BaTiO₃ photoelectrode led to improved photovoltaic performance. Additionally, doping TiO₂ with elements like iron (Fe) has been found to enhance the material’s surface area and electronic properties.
The incorporation of LSPRM, such as silver or gold nanoparticles, has been extensively investigated to enhance light absorption and facilitate faster electron injection in DSSCs. These nanoparticles generate strong localized surface plasmon resonance (LSPR) fields, which can significantly enhance the photonic absorption of dyes. In the future, the composition and construction of the thin layer system, elemental doping, and the addition of LSPRM to HS TiO2-based photoanode will become promising studies in developing more efficient DSSC.
Credit authorship contribution statement
Khusna Arif Rakhman: Data curation, Investigation, Methodology, Writing – original draft. Nurul Hidayat Aprilita: Formal analysis, Supervision, Writing – review & editing. Rohana Adnan: Formal analysis, Methodology, Writing – review & editing. Indriana Kartini: Funding acquisition, Methodology, Supervision, Writing – review & editing.
Declaration of competing interest
The authors declare that they have no known financial interests in the work reported in this paper.
Declaration of generative AI and AI-assisted technologies in the writing process
The authors confirm that there was no use of artificial intelligence (AI)-assisted technology for assisting in the writing or editing of the manuscript and no images were manipulated using AI.
References
- Enhanced photovoltaic properties of dye-sensitized solar cell based on ultrathin 2D TiO2 nanostructures. Applied Surface Science. 2016;368:403-8. https://doi.org/10.1016/j.apsusc.2016.02.010
- [Google Scholar]
- Trilayered photoanode of TiO2 nanoparticles on a 1D-3D nanostructured TiO2-grown flexible Ti substrate for high-efficiency (9.1%) dye-sensitized solar cells with unprecedentedly high photocurrent density. The Journal of Physical Chemistry C. 2014;118:16426-16432. https://doi.org/10.1021/jp4116782
- [Google Scholar]
- Enhanced photovoltaic properties in dye sensitized solar cells by surface treatment of SnO2 photoanodes. Scientific Reports. 2016;6:23312. https://doi.org/10.1038/srep23312
- [Google Scholar]
- Fabrication, optimization and characterization of natural dye sensitized solar cell. Scientific Reports. 2017;7:41470. https://doi.org/10.1038/srep41470
- [Google Scholar]
- Electron transport properties analysis of titanium dioxide dye-sensitized solar cells (TiO2-DSSCs) based natural dyes using electrochemical impedance spectroscopy concept: A review. Solar Energy. 2020;207:1088-1121. https://doi.org/10.1016/j.solener.2020.07.028
- [Google Scholar]
- Nanostructured photoanode and counter electrode materials for efficient dye-sensitized solar cells (DSSCs) Solar Energy. 2019;185:165-188. https://doi.org/10.1016/j.solener.2019.04.057
- [Google Scholar]
- Dye-sensitized solar cells based on natural photosensitizers: A green view from Iran. Journal of Alloys and Compounds. 2020;828:154329. https://doi.org/10.1016/j.jallcom.2020.154329
- [Google Scholar]
- Photocurrent generation by adsorption of two main pigments of halobacterium salinarum on TiO2 nanostructured electrode. Biotechnology and Applied Biochemistry. 2015;62:121-5. https://doi.org/10.1002/bab.1244
- [Google Scholar]
- Fabrication of dye‐Sensitized solar cells based on novel mixed tungsten–Molybdenum and tungsten–Titanium oxides nanoparticles prepared by impulse underwater plasma. Physica Status Solidi (a). 2023;220:2200435.. https://doi.org/10.1002/pssa.202200435
- [Google Scholar]
- Phthaloylchitosan-based gel polymer electrolytes for efficient dye-sensitized solar cells. Journal of Chemistry. 2014;2014:1-8. https://doi.org/10.1155/2014/783023
- [Google Scholar]
- Dye-sensitized solar cell employing chitosan-based biopolymer electrolyte. IOP Conference Series: Materials Science and Engineering. 2023;1291:012014. https://doi.org/10.1088/1757-899x/1291/1/012014
- [Google Scholar]
- On the plasmonic photovoltaic. ACS Nano. 2014;8:6066-6073. https://doi.org/10.1021/nn501379r
- [Google Scholar]
- Simple preparation of fluorine-doped TiO₂ photoanode for high performance dye sensitized solar cells. Electrochimica Acta. 2015;173:834-8. https://doi.org/10.1016/j.electacta.2015.05.118
- [Google Scholar]
- Improved electron transfer of TiO2 based dye sensitized solar cells using Ge as sintering aid. Optik. 2018;157:134-140. https://doi.org/10.1016/j.ijleo.2017.11.073
- [Google Scholar]
- Titanium dioxide nanomaterials for photovoltaic applications. Chemical Reviews. 2014;114:10095-10130. https://doi.org/10.1021/cr400606n
- [Google Scholar]
- Properties of chromophores determining recombination at the TiO2–dye–electrolyte interface. Langmuir. 2013;29:8773-8781.
- [Google Scholar]
- Engineering flexible dye-sensitized solar cells for portable electronics. Solar Energy. 2019;177:80-98. https://doi.org/10.1016/j.solener.2018.11.017
- [Google Scholar]
- Nano-structured TiO2/ZnO nanocomposite for dye-sensitized solar cells application: A review. Renewable and Sustainable Energy Reviews. 2018;81:2264-2270. https://doi.org/10.1016/j.rser.2017.06.035
- [Google Scholar]
- Nanostructured photoanode materials and their deposition methods for efficient and economical third generation dye-sensitized solar cells: A comprehensive review. Renewable and Sustainable Energy Reviews. 2020;129:109919. https://doi.org/10.1016/j.rser.2020.109919
- [Google Scholar]
- Optimized TiO2 nanoparticle packing for DSSC photovoltaic applications. Solar Energy Materials and Solar Cells. 2016;148:52-9. https://doi.org/10.1016/j.solmat.2015.09.010
- [Google Scholar]
- Particle size effects of TiO2 layers on the solar efficiency of dye-sensitized solar cells. International Journal of Photoenergy 2013:2013.
- [Google Scholar]
- TiO2 nanostructures with controlled morphology for improved electrical properties of photoanodes and quantum dot sensitized solar cell characteristics. Surfaces and Interfaces. 2019;17:100350. https://doi.org/10.1016/j.surfin.2019.100350
- [Google Scholar]
- Seed-mediated photodeposition route to ag-decorated SiO2@TiO2 microspheres with ideal core-shell structure and enhanced photocatalytic activity. Applied Surface Science. 2018;434:1007-1014. https://doi.org/10.1016/j.apsusc.2017.11.020
- [Google Scholar]
- Effect of particle size on the performance of TiO2 based dye-sensitized solar cells. Chemistry Select. 2018;3:9872-9880. https://doi.org/10.1002/slct.201801745
- [Google Scholar]
- Enhanced performances of dye-sensitized solar cells based on Au-TiO2 and Ag-TiO2 plasmonic hybrid nanocomposites. Applied Surface Science. 2018;430:415-423. https://doi.org/10.1016/j.apsusc.2017.07.107
- [Google Scholar]
- Dye-sensitized solar cells based on surficial TiO2 modification. Solar Energy. 2019;184:454-465. https://doi.org/10.1016/j.solener.2019.04.032
- [Google Scholar]
- Recent progress in dye sensitized solar cell materials and photo-supercapacitors: A review. Journal of Power Sources. 2021;493:229698. https://doi.org/10.1016/j.jpowsour.2021.229698
- [Google Scholar]
- Effect of TiO2 particle and pore size on DSSC efficiency. Materials for Renewable and Sustainable Energy. 2020;9 https://doi.org/10.1007/s40243-020-00173-7
- [Google Scholar]
- Pure and highly Nb-doped titanium dioxide nanotubular arrays: Characterization of local surface properties. Nanomaterials (Basel, Switzerland). 2017;7:456. https://doi.org/10.3390/nano7120456
- [Google Scholar]
- Fabrication of TiO2 hollow microspheres assembly from nanosheets (TiO2-HMSs-NSs) with enhanced photoelectric conversion efficiency in DSSCs and photocatalytic activity. Applied Catalysis B: Environmental. 2017;210:184-193. https://doi.org/10.1016/j.apcatb.2017.03.064
- [Google Scholar]
- Metal oxides for dye-sensitized solar cells. In: Metal Oxides for next-generation optoelectronic, photonic, and photovoltaic applications. Elsevier; 2024. p. :543-576. https://doi.org/10.1016/B978-0-323-99143-8.00017-1
- [Google Scholar]
- Contribution of multiple reflections to light utilization efficiency of submicron hollow TiO2 photocatalyst. Science China Materials. 2016;59:1017-1026. https://doi.org/10.1007/s40843-016-5127-7
- [Google Scholar]
- Au/TiO2 nanotube array based multi-hierarchical architecture for highly efficient dye-sensitized solar cells. Journal of Power Sources. 2019;439:227076. https://doi.org/10.1016/j.jpowsour.2019.227076
- [Google Scholar]
- Au nanoparticle-decorated urchin-like TiO2 hierarchical microspheres for high performance dye-sensitized solar cells. Superlattices and Microstructures. 2019;129:185-192. https://doi.org/10.1016/j.spmi.2019.03.028
- [Google Scholar]
- Au nanoparticle-decorated urchin-like TiO2 hierarchical microspheres for high performance dye-sensitized solar cells. Electrochimica Acta. 2019;293:230-9. https://doi.org/10.1016/j.electacta.2018.10.035
- [Google Scholar]
- Fabrication of Au@Ag core/shell nanoparticles decorated TiO2 hollow structure for efficient light-harvesting in dye-sensitized solar cells. ACS Applied Materials & Interfaces. 2015;7:2055-2063. https://doi.org/10.1021/am508065n
- [Google Scholar]
- Development of free-standing titanium dioxide hollow nanofibers photocatalyst with enhanced recyclability. Membranes. 2022;12:342. https://doi.org/10.3390/membranes12030342
- [Google Scholar]
- Hollow nanostructures for photocatalysis: Advantages and challenges. Advanced materials (Deerfield Beach, Fla.). 2019;31:e1801369. https://doi.org/10.1002/adma.201801369
- [Google Scholar]
- Design of heterostructured hollow photocatalysts for solar‐to‐chemical energy conversion. Advanced Materials. 2019;31 https://doi.org/10.1002/adma.201900281
- [Google Scholar]
- Construction of hierarchical hollow Co9 S8 /ZnIn2 S4 tubular heterostructures for highly efficient solar energy conversion and environmental remediation. Angewandte Chemie (International ed. in English). 2020;59:8255-8261. https://doi.org/10.1002/anie.202000503
- [Google Scholar]
- Hollow semiconductor photocatalysts for solar energy conversion. Advanced Powder Materials. 2022;1:100021. https://doi.org/10.1016/j.apmate.2021.11.008
- [Google Scholar]
- Towards sustainable solar cells: Unveiling the latest developments in bio-nano materials for enhanced DSSC efficiency. Clean Energy. 2024;8:238-257. https://doi.org/10.1093/ce/zkae031
- [Google Scholar]
- A review on the current status of dye‐sensitized solar cells: Toward sustainable energy. Energy Science & Engineering. 2024;12:3188-3226.
- [Google Scholar]
- Accelerating directional charge separation via built-in interfacial electric fields originating from work-function differences. Chinese Journal of Catalysis. 2021;42:583-594. https://doi.org/10.1016/s1872-2067(20)63649-x
- [Google Scholar]
- Hierarchical co–Pi clusters/Fe2O3 nanorods/FTO micropillars 3D branched photoanode for high-performance photoelectrochemical water splitting. Nanomaterials. 2022;12:3664. https://doi.org/10.3390/nano12203664
- [Google Scholar]
- Porous versus compact hematite nanorod photoanode for high-performance photoelectrochemical water oxidation. ACS Sustainable Chemistry & Engineering. 2019;7:11377-11385. https://doi.org/10.1021/acssuschemeng.9b01045
- [Google Scholar]
- Advancements in the development of TiO2 photoanodes and its fabrication methods for dye sensitized solar cell (DSSC) applications . A review. Renewable and Sustainable Energy Reviews. 2017;77:89-108. https://doi.org/10.1016/j.rser.2017.03.129
- [Google Scholar]
- Recent progresses in solar cells: Insight into hollow micro/nano–structures. Renewable and Sustainable Energy Reviews. 2016;64:543-568. https://doi.org/10.1016/j.rser.2016.06.028
- [Google Scholar]
- Self-templated synthesis of large-scale hierarchical anatase titania nanotube arrays on transparent conductive substrate for dye-sensitized solar cells. Advanced Powder Technology. 2019;30:572-580. https://doi.org/10.1016/j.apt.2018.12.010
- [Google Scholar]
- Microporous and mesoporous materials synergistic effects of inter- and intra-particle porosity of TiO2 nanoparticles on photovoltaic performance of dye-sensitized solar cells. Microporous and Mesoporous Materials. 2018;266:214-222. https://doi.org/10.1016/j.micromeso.2018.03.003
- [Google Scholar]
- Preparation of hierarchical rutile TiO2 microspheres as scattering centers for efficient dye-sensitized solar cells. Electrochimica Acta. 2017;255:187-194. https://doi.org/10.1016/j.electacta.2017.09.158
- [Google Scholar]
- A review of hierarchical nanostructures of TiO2: Advances and applications. Applied Surface Science Advances. 2021;3:100063.
- [Google Scholar]
- Hierarchical nanostructures for energy devices. The Royal Society of Chemistry 2014 https://doi.org/10.1016/j.apsadv.2021.100063
- [Google Scholar]
- Hierarchical TiO2 microspheres composed with nanoparticle-decorated nanorods for the enhanced photovoltaic performance in dye-sensitized solar cells. RSC Advances. 2019;9:3056-3062. https://doi.org/10.1039/c8ra09145e
- [Google Scholar]
- Scattering resonance enhanced dye absorption of dye sensitized solar cells at optimized hollow structure size. Journal of Power Sources. 2014;268:1-6. https://doi.org/10.1016/j.jpowsour.2014.06.015
- [Google Scholar]
- Structural regulation of hollow spherical TiO2 by varying titanium source amount and their thermal insulation property. Colloids and Surfaces A: Physicochemical and Engineering Aspects. 2018;537:69-75. https://doi.org/10.1016/j.colsurfa.2017.10.019
- [Google Scholar]
- Biotemplated synthesis of 3D rare earth–doped TiO2 hollow spheres for photocatalytic application. Journal of Physics and Chemistry of Solids. 2019;126:78-84. https://doi.org/10.1016/j.jpcs.2018.10.023
- [Google Scholar]
- Titanium dioxide nanomaterials: Synthesis, properties, modifications and applications. Chemical Reviews. 2007;107:2891-2959. https://doi.org/10.1021/cr0500535
- [Google Scholar]
- Hollowing sn-doped TiO2 nanospheres via ostwald ripening. Journal of the American Chemical Society. 2007;129:15839-15847. https://doi.org/10.1021/ja073521w
- [Google Scholar]
- The synthesis and mechanism exploration of europium-doped LiYF4 micro-octahedron phosphors with multilevel interiors. Dalton Transactions (Cambridge, England: 2003). 2014;43:5453-5461. https://doi.org/10.1039/c3dt53087f
- [Google Scholar]
- One-Dimensional Titanium Dioxide Nanomaterials: Nanowires. Chemical Reviews. 2014;114:9346-9384. https://doi.org/10.1021/cr400633s
- [Google Scholar]
- Solvothermal synthesis of TiO2 hollow nanospheres utilizing the kirkendall effect and their photocatalytic activities. Applied Surface Science. 2014;321:86-93. https://doi.org/10.1016/j.apsusc.2014.09.197
- [Google Scholar]
- Solvothermal synthesis of mesoporous TiO2 microspheres and their excellent photocatalytic performance under simulated sunlight irradiation. Solid State Sciences. 2013;20:8-14. https://doi.org/10.1016/j.solidstatesciences.2013.02.026
- [Google Scholar]
- Revealing bismuth oxide hollow nanoparticle formation by the kirkendall effect. Nano Letters. 2013;13:5715-5719. https://doi.org/10.1021/nl4035362
- [Google Scholar]
- Formation of nanotubes and hollow nanoparticles based on kirkendall and diffusion processes: A review. Small (Weinheim an der Bergstrasse, Germany). 2007;3:1660-1671. https://doi.org/10.1002/smll.200700382
- [Google Scholar]
- A review on hollow nanostructures, its derived composites: Preparation, structural control, alterations, and their utilization as an electrode for supercapacitors, rechargeable batteries and electrocatalysis. Journal of Energy Storage. 2024;98:113143. https://doi.org/10.1016/j.est.2024.113143
- [Google Scholar]
- Their lithium storage and photocatalytic properties. Chemical Physics. 2019;517:222-7. https://doi.org/10.1016/j.chemphys.2018.10.022
- [Google Scholar]
- Improved utilization of photogenerated charge using fluorine-doped TiO2 hollow spheres scattering layer in dye-sensitized solar cells. ACS Applied Materials & Interfaces. 2012;4:3712-7. https://doi.org/10.1021/am300801f
- [Google Scholar]
- Preparation of TiO2 hollow microspheres by a novel vesicle template method and their enhanced photocatalytic properties. Ceramics International. 2013;39:9465-9470. https://doi.org/10.1016/j.ceramint.2013.05.064
- [Google Scholar]
- Uniform mesoporous anatase−brookite biphase TiO2 hollow sphere with high crystallinity via ostwald ripening. The Journal of Physical Chemistry C. 2013;117:21718-21723. https://doi.org/10.1021/jp408322g
- [Google Scholar]
- Uniform hollow TiO2:Sm3+ spheres: Solvothermal synthesis and luminescence properties. Powder Technology. 2013;239:403-8. https://doi.org/10.1016/j.powtec.2013.02.010
- [Google Scholar]
- Effects of size and shell thickness of TiO2 hierarchical hollow spheres on photocatalytic behavior: An experimental and theoretical study. Applied Catalysis B: Environmental. 2014;147:499-507. https://doi.org/10.1016/j.apcatb.2013.09.033
- [Google Scholar]
- One-step template-free fabrication of mesoporous ZnO/TiO2 hollow microspheres with enhanced photocatalytic activity. Applied Surface Science. 2014;307:263-271. https://doi.org/10.1016/j.apsusc.2014.04.023
- [Google Scholar]
- TiO2 hollow microspheres with mesoporous surface: Superior adsorption performance for dye removal. Applied Surface Science. 2014;305:352-8. https://doi.org/10.1016/j.apsusc.2014.03.089
- [Google Scholar]
- Au/TiO2 hollow spheres with synergistic effect of plasmonic enhancement and light scattering for improved dye-sensitized solar cells. ACS Applied Materials & Interfaces. 2017;9:31691-8. https://doi.org/10.1021/acsami.7b04624
- [Google Scholar]
- The high surface energy of NiO {110} facets incorporated into TiO2 hollow microspheres by etching Ti plate for enhanced photocatalytic and photoelectrochemical activity. Applied Surface Science. 2017;396:1539-1545. https://doi.org/10.1016/j.apsusc.2016.11.205
- [Google Scholar]
- Non-aqueous preparation of anatase TiO2 hollow microspheres for efficient dye-sensitized solar cells. Advanced Powder Technology. 2019;30:2408-2415. https://doi.org/10.1016/j.apt.2019.07.023
- [Google Scholar]
- Facile template-free route to synthesis visible light respensive hollow TiO2 spheres. Materials Letters. 2019;244:50-3. https://doi.org/10.1016/j.matlet.2019.02.055
- [Google Scholar]
- Facile one-pot synthesis of surface-fluorinated TiO2 hollow spheres with enhanced photocatalytic activity. Journal of Photochemistry and Photobiology A: Chemistry. 2020;400:112654. https://doi.org/10.1016/j.jphotochem.2020.112654
- [Google Scholar]
- Preparation and electrorheological properties of eggshell-like TiO2 hollow spheres via one step template-free solvothermal method. Colloids and Surfaces A: Physicochemical and Engineering Aspects. 2020;601:125055. https://doi.org/10.1016/j.colsurfa.2020.125055
- [Google Scholar]
- Solvent-controlled synthesis and photocatalytic activity of hollow TiO2 microspheres prepared by the solvothermal method. Colloids and Surfaces A: Physicochemical and Engineering Aspects. 2022;633:127931. https://doi.org/10.1016/j.colsurfa.2021.127931
- [Google Scholar]
- Preparation of mesoscale hollow spheres of TiO2 and SnO2 by templating against crystalline arrays of polystyrene beads. Advanced Materials. 2000;12:206-9. https://doi.org/10.1002/(sici)1521-4095(200002)12:3<206::aid-adma206>3.0.co;2-5
- [Google Scholar]
- Novel and facile method for the preparation of monodispersed titania hollow spheres. Langmuir: The ACS Journal of Surfaces and Colloids. 2006;22:3858-3863. https://doi.org/10.1021/la0534221
- [Google Scholar]
- Preparation of submicrometer-sized titania hollow spheres by templating sulfonated polystyrene latex particles. Materials Letters. 2007;61:1572-5. https://doi.org/10.1016/j.matlet.2006.07.081
- [Google Scholar]
- Fabrication of hollow hybrid microspheres coated with silica/titania via sol-gel process and enhanced photocatalytic activities. The Journal of Physical Chemistry C. 2007;111:8180-7.
- [Google Scholar]
- Fabrication of hollow titania microspheres with tailored shell thickness. Colloid and Polymer Science. 2008;286:593-601. https://doi.org/10.1007/s00396-007-1833-3
- [Google Scholar]
- Performance of tin doped titania hollow spheres as electrocatalysts for hydrogen and oxygen production in water electrolysis. International Journal of Hydrogen Energy. 2008;33:3270-3280. https://doi.org/10.1016/j.ijhydene.2008.03.057
- [Google Scholar]
- Preparation of TiO2 hollow spheres for DSSC photoanodes. Journal of Physics and Chemistry of Solids. 2014;75:38-41. https://doi.org/10.1016/j.jpcs.2013.08.005
- [Google Scholar]
- Efficient dye-sensitized solar cells based on carbon-doped TiO2 hollow spheres and nanoparticles. Journal of Materials Science: Materials in Electronics. 2015;26:8863-8876. https://doi.org/10.1007/s10854-015-3567-1
- [Google Scholar]
- Monodisperse hollow TiO₂ spheres for thermal insulation materials: Template-free synthesis, characterization and properties. Ceramics International. 2017;43:8596-8602. https://doi.org/10.1016/j.ceramint.2017.03.155
- [Google Scholar]
- Polydopamine-induced fabrication of Ag-TiO2 hollow nanospheres and their application in visible-light photocatalysis. Colloids and Surfaces A: Physicochemical and Engineering Aspects. 2020;586:124283. https://doi.org/10.1016/j.colsurfa.2019.124283
- [Google Scholar]
- Size controllable synthesis and photocatalytic performance of mesoporous TiO2 hollow spheres. Journal of Materials Science & Technology. 2020;48:105-113. https://doi.org/10.1016/j.jmst.2020.03.013
- [Google Scholar]
- Design and electrospun closed cell structured SiO2 nanocomposite fiber by hollow SiO2/TiO2 spheres for thermal insulation. Composites Science and Technology. 2022;218:109152. https://doi.org/10.1016/j.compscitech.2021.109152
- [Google Scholar]
- Cellular uptake, cytotoxicity, and innate immune response of silica-titania hollow nanoparticles based on size and surface functionality. ACS Nano. 2010;4:5301-13. https://doi.org/10.1021/nn100561e
- [Google Scholar]
- Synthesis of titania embedded silica hollow nanospheres via sonication mediated etching and re-deposition. Chemical communications (Cambridge, England). 2011;47:7092-4. https://doi.org/10.1039/c1cc11185j
- [Google Scholar]
- Characterizing size and porosity of hollow nanoparticles: SAXS, SANS, TEM, DLS, and adsorption isotherms compared. Langmuir: The ACS Journal of Surfaces and Colloids. 2012;28:15350-15361. https://doi.org/10.1021/la302236u
- [Google Scholar]
- Synthesis of the double-shell anatase-rutile TiO2 hollow spheres with enhanced photocatalytic activity. Nanoscale. 2013;5:12150-12155. https://doi.org/10.1039/c3nr04043g
- [Google Scholar]
- SiO2-TiO2 hollow nanoparticles decorated with Ag nanoparticles: enhanced visible light absorption and improved light scattering in dye‐sensitized solar cells. Chemistry. 2014;20:4439-4446. https://doi.org/10.1002/chem.201304522
- [Google Scholar]
- Synthesis of TiO2 hollow spheres by selective etching of Au@TiO2 core-shell nanoparticles for dye sensitized solar cell applications. RSC Advances. 2014;4:3529-3935. https://doi.org/10.1039/C3RA45860A
- [Google Scholar]
- Fabrication of TiO2 hollow microspheres using K3PW12O40 as template. Chinese Journal of Catalysis. 2015;36:2237-2243. https://doi.org/10.1016/s1872-2067(15)60987-1
- [Google Scholar]
- SiO2/TiO2 double-shell hollow particles: Fabrication and UV-Vis spectrum characterization. Advanced Powder Technology. 2016;27:812-828. https://doi.org/10.1016/j.apt.2015.10.016
- [Google Scholar]
- Synthesis and electrophoretic deposition of hollow-TiO2 nanoparticles for dye sensitized solar cell applications. Journal of Alloys and Compounds. 2016;672:212-222. https://doi.org/10.1016/j.jallcom.2016.02.164
- [Google Scholar]
- Hollow titania spheres loaded with noble metal nanoparticles for photocatalytic water oxidation. Microporous and Mesoporous Materials. 2018;264:147-150. https://doi.org/10.1016/j.micromeso.2018.01.012
- [Google Scholar]
- Synthesis of hierarchical silica/Titania hollow nanoparticles and their enhanced electroresponsive activity. ACS Applied Materials & Interfaces. 2018;10:6570-9. https://doi.org/10.1021/acsami.7b18895
- [Google Scholar]
- Mie resonance in hollow nanoshells of ternary TiO2-Au-CdS and enhanced photocatalytic hydrogen evolution. Applied Catalysis B: Environmental. 2020;276:119153. https://doi.org/10.1016/j.apcatb.2020.119153
- [Google Scholar]
- Synthesis of TiO2 hollow nanoparticles with different shell thickness and effect of structure on photocatalytic activity. Solid State Sciences. 2020;103:106179. https://doi.org/10.1016/j.solidstatesciences.2020.106179
- [Google Scholar]
- TiO2 hollow heterophase junction with enhanced pollutant adsorption, light harvesting, and charge separation for photocatalytic degradation of volatile organic compounds. Chemical Engineering Journal. 2020;391:123602. https://doi.org/10.1016/j.cej.2019.123602
- [Google Scholar]
- Synthesis of hollow TiO2@SiO2 spheres via a recycling template method for solar heat protection coating. Ceramics International. 2021;47:2678-2685. https://doi.org/10.1016/j.ceramint.2020.09.117
- [Google Scholar]
- Colloidal crystallite suspensions studied by high pressure small angle x-ray scattering. The Journal of Chemical Physics. 2016;144:084903. https://doi.org/10.1063/1.4941563
- [Google Scholar]
- Self-development of hollow TiO₂ nanoparticles by chemical conversion coupled with ostwald ripening. Chemical Engineering Journal. 2016;286:266-271. https://doi.org/10.1016/j.cej.2015.10.077
- [Google Scholar]
- Facile fabrication of robust polydopamine microcapsules for insulin delivery. Journal of Colloid and Interface Science. 2017;487:12-9. https://doi.org/10.1016/j.jcis.2016.10.012
- [Google Scholar]
- Preparation and characterization of low density polystyrene/TiO2 core-shell particles for electronic paper application. Current Applied Physics. 2009;9:755-9. https://doi.org/10.1016/j.cap.2008.07.011
- [Google Scholar]
- Controllable Synthesis and Crystallization of Nanoporous TiO2 Deep-Submicrospheres and Nanospheres via an Organic Acid-Mediated Sol−Gel Process. Langmuir. 2020;36:7447-7555. https://doi.org/10.1021/acs.langmuir.0c01008
- [Google Scholar]
- Nitrogen-doped SiO2/TiO2 core/shell nanoparticles as highly efficient visible light photocatalyst. Catalysis Communications. 2010;11:378-382. https://doi.org/10.1016/j.catcom.2009.11.005
- [Google Scholar]
- Effect of annealing and etching times on anatase TiO2 hollow sphere. Molekul. 2023;18:273. https://doi.org/10.20884/1.jm.2023.18.2.7480
- [Google Scholar]
- One-pot synthesis of hollow sphere TiO2/Ag nanoparticles co-sensitized with peonidin: Pelargonidin for dye-sensitized solar cells applications. Journal of Materials Science: Materials in Electronics. 2024;35:1926. https://doi.org/10.1007/s10854-024-13693-z
- [Google Scholar]
- The evolution of ‘sol–gel’ chemistry as a technique for materials synthesis. Materials Horizons. 2016;3:91-112. https://doi.org/10.1039/C5MH00260E
- [Google Scholar]
- Different methods used for the synthesis of TiO2 based nanomaterials: A review. American Journal of Nano Research and Applications. 2018;6:1. https://doi.org/10.11648/j.nano.20180601.11
- [Google Scholar]
- Double light-scattering layer film based on TiO2 hollow spheres and TiO2 nanosheets: Improved efficiency in dye-sensitized solar cells. Journal of Alloys and Compounds. 2013;575:168-173. https://doi.org/10.1016/j.jallcom.2013.02.045
- [Google Scholar]
- Facile two-step preparation of polystyrene/anatase TiO2 core/shell colloidal particles and their potential use as an oxidation photocatalyst. Materials Chemistry and Physics. 2014;144:498-504. https://doi.org/10.1016/j.matchemphys.2014.01.026
- [Google Scholar]
- Formation of core/shell structured polystyrene/anatase TiO2 photocatalyst via vapor phase hydrolysis. Applied Catalysis B: Environmental. 2012;123-124:123-133. https://doi.org/10.1016/j.apcatb.2012.04.032
- [Google Scholar]
- Designed synthesis of SiO2/TiO2 core/shell structure as light scattering material for highly efficient dye-sensitized solar cells. ACS Applied Materials & Interfaces. 2013;5:4815-4820. https://doi.org/10.1021/am400441v
- [Google Scholar]
- A facile template-free route for synthesis of anatase TiO2 hollow spheres for dye-sensitized solar cells. Electrochimica Acta. 2014;143:129-134. https://doi.org/10.1016/j.electacta.2014.07.106
- [Google Scholar]
- Template-assisted hydrothermal synthesis and photocatalytic activity of novel TiO2 hollow nanostructures. Ceramics International. 2013;39:4969-4974. https://doi.org/10.1016/j.ceramint.2012.11.093
- [Google Scholar]
- Thickness effects on light absorption and scattering for nanoparticles in the shape of hollow spheres. The Journal of Physical Chemistry C. 2015;119:25754-25760. https://doi.org/10.1021/acs.jpcc.5b08435
- [Google Scholar]
- Preparation and characterization of SiO2/TiO2 core-shell particles with controlled shell thickness. Materials Chemistry and Physics. 2007;106:39-44. https://doi.org/10.1016/j.matchemphys.2007.05.019
- [Google Scholar]
- Enhanced light scattering and photovoltaic performance for dye-sensitized solar cells by embedding submicron SiO2/TiO2 core/shell particles in photoanode. Ceramics International. 2013;39:5407-5413. https://doi.org/10.1016/j.ceramint.2012.12.048
- [Google Scholar]
- Fabrication of SiO2/TiO2 double-shelled hollow nanospheres with controllable size via Sol − Gel reaction and sonication-mediated etching. ACS Applied Materials & Interfaces. 2014;6:15420-12426. https://doi.org/10.1021/am503957f
- [Google Scholar]
- Enhanced electroresponse of alkaline earth metal-doped Silica/Titania spheres by synergetic effect of dispersion stability and dielectric property. ACS Applied Materials & Interfaces. 2015;7:18977-18984. https://doi.org/10.1021/acsami.5b02388
- [Google Scholar]
- Enhanced photocatalytic properties of core@shell SiO2@TiO2 nanoparticles. Applied Catalysis B: Environmental. 2015;179:333-343. https://doi.org/10.1016/j.apcatb.2015.05.036
- [Google Scholar]
- Photocatalytic and photoluminescence properties of core−shell SiO2@TiO2:Eu3+,Sm3+ and its etching products. ACS Sustainable Chemistry & Engineering. 2018;6:223-236. https://doi.org/10.1021/acssuschemeng.7b02285
- [Google Scholar]
- SiO2@TiO2:Eu3+ and its derivatives: Design, synthesis, and properties. Crystal Growth & Design. 2017;17:6486−6497. https://doi.org/10.1021/acs.cgd.7b01149
- [Google Scholar]
- Development of SiO2@TiO2 core-shell nanospheres for catalytic applications. Applied Surface Science. 2018;441:223-231. https://doi.org/10.1016/j.apsusc.2018.02.008
- [Google Scholar]
- A tough and fluorescent dual nanocomposite hydrogel based on SiO2@TiO2 core-shell nanoparticles. Applied Surface Science. 2019;467-468:588-595. https://doi.org/10.1016/j.apsusc.2018.10.208
- [Google Scholar]
- Large-scale preparation of rice-husk-derived mesoporous SiO2@TiO2 as efficient and promising photocatalysts for organic contaminants degradation. Applied Surface Science. 2019;467-468:1187-1194. https://doi.org/10.1016/j.apsusc.2018.10.275
- [Google Scholar]
- Solvent-controlled deposition of titania on silica spheres for the preparation of SiO2@TiO2 core@shell nanoparticles with enhanced photocatalytic activity. Colloids and Surfaces A: Physicochemical and Engineering Aspects. 2019;570:293-305. https://doi.org/10.1016/j.colsurfa.2019.03.036
- [Google Scholar]
- SiO2@TiO2 core@Shell nanoparticles deposited on 2D-layered ZnIn2S4 to form a ternary heterostructure for simultaneous photocatalytic hydrogen production and organic pollutant degradation. Inorganic Chemistry. 2020;59:2278-2287. https://doi.org/10.1021/acs.inorgchem.9b03007
- [Google Scholar]
- TiO2 hollow spheres as light scattering centers in TiO2 photoanodes for dye-sensitized solar cells: The effect of sphere diameter. Journal of Alloys and Compounds. 2016;663:211-216. https://doi.org/10.1016/j.jallcom.2015.12.118
- [Google Scholar]
- Electrochimica Acta Anatase TiO2 hollow spheres with small dimension fabricated via a simple preparation method for dye-sensitized solar cells with an ionic liquid electrolyte. Electrochim Acta. 2012;60:422-7. https://doi.org/10.1016/j.electacta.2011.11.088
- [Google Scholar]
- Dye-sensitized solar cells based on TiO2 hollow spheres/TiO2 nanotube array composite films. Applied Surface Science. 2014;309:85-9. https://doi.org/10.1016/j.apsusc.2014.04.184
- [Google Scholar]
- Dye-sensitized solar cells based on anatase TiO2 hollow spheres/carbon nanotube composite films. Journal of Power Sources. 2011;196:7891-8. https://doi.org/10.1016/j.jpowsour.2011.05.014
- [Google Scholar]
- Synthesis and characterization of Fe-doped TiO2 hollow spheres for dye-sensitized solar cell applications. Materials Science and Engineering: B. 2021;271:115311. https://doi.org/10.1016/j.mseb.2021.115311
- [Google Scholar]
- Improvement of the photoelectric dye sensitized solar cell performance using Fe/S–TiO2 nanoparticles as photoanode electrode. Scientific Reports. 2024;14 https://doi.org/10.1038/s41598-024-54895-z
- [Google Scholar]
- Preparation of plasmonic monolayer with Ag and Au nanoparticles for dye-sensitized solar cells. Chemical Physics Letters. 2017;687:152-7. https://doi.org/10.1016/j.cplett.2017.08.051
- [Google Scholar]
- Plasmonic mesoporous core-shell Ag-Au@TiO2 photoanodes for efficient light harvesting in dye sensitized solar cells. Solar Energy. 2019;193:820-827. https://doi.org/10.1016/j.solener.2019.10.039
- [Google Scholar]
- Know thy nano neighbor Plasmonic versus electron charging effects of metal nanoparticles in dye-sensitized solar cells. ACS Nano. 2012;6:4418-4427. https://doi.org/10.1021/nn301137r
- [Google Scholar]
- Hybrid MoS2-gap-mode metasurface photodetectors. Journal of Physics D: Applied Physics. 2019;52:374001. https://doi.org/10.1088/1361-6463/ab2aba
- [Google Scholar]
- Enhanced photovoltaic performance of silver@titania plasmonic photoanode in dye-sensitized solar cells. RSC Advances. 2014;4:38111-8. https://doi.org/10.1039/c4ra05689b
- [Google Scholar]
- Distance and plasmon wavelength dependent fluorescence of molecules bound to silica-coated gold nanorods. ACS Nano. 2014;8:8392-8406. https://doi.org/10.1021/nn502887j
- [Google Scholar]
- Dual‐Confined flexible sulfur cathodes encapsulated in nitrogen‐Doped double‐Shelled hollow carbon spheres and wrapped with graphene for Li–S batteries. Advanced Energy Materials. 2015;5:1402263. https://doi.org/10.1002/aenm.201402263
- [Google Scholar]
- Scalable synthesis of mesoporous TiO2 for environmental photocatalytic applications. Materials (Basel, Switzerland). 2019;12:1853. https://doi.org/10.3390/ma12111853
- [Google Scholar]